- 1Center for Tree Science, The Morton Arboretum, Lisle, IL, United States
- 2Canopy Watch International, Boise, ID, United States
- 3Ecology and Evolutionary Biology, University of Michigan, Ann Arbor, MI, United States
- 4School of Biological Sciences, University of Aberdeen, Aberdeen, United Kingdom
- 5Department of Mechanical Engineering, Université de Sherbrooke, Sherbrooke, QC, Canada
- 6Department of Biological Sciences, DePaul University, Chicago, IL, United States
- 7Department of Biology, University of Louisville, Louisville, KY, United States
- 8Department of Biology, Washington University in Saint Louis, Saint Louis, MO, United States
- 9UK Centre for Ecology & Hydrology, Penicuik, United Kingdom
- 10Instituto de Investigaciones de la Amazonia Peruana (IIAP), Iquitos, Peru
- 11Laboratory of Geo-Information Science and Remote Sensing, Wageningen University and Research, Wageningen, Netherlands
- 12School of Ecology and Environmental Science, Yunnan University, Kunming, China
- 13Department of Botany, University of Tartu, Tartu, Estonia
- 14Geosciences and Natural Resources Department, Western Carolina University, Cullowhee, NC, United States
- 15National Ecological Observatory Network, Battelle, Boulder, CO, United States
- 16Grupo de Investigación en Biodiversidad, Medio Ambiente y Salud-BIOMAS- Universidad de las Américas, Quito, Ecuador
- 17Instituto de Biología, Universidad Nacional Autónoma de México, Mexico City, Mexico
- 18Myerscough College, Preston, United Kingdom
- 19Biodiversity Monitoring and Assessment, Royal Belgian Institute of Natural Sciences, and Evolutionary Biology, and Ecology and Université Libre de Bruxelles, Brussels, Belgium
- 20Department of Biological Sciences, National University of Singapore, Singapore, Singapore
- 21TREE Foundation, Sarasota, FL, United States
- 22Department of Anthropology, Department of Ecology and Evolutionary Biology, Program in the Environment, and School for Environment and Sustainability, University of Michigan, Ann Arbor, MI, United States
- 23Department of Botany and Biodiversity Research Centre, The University of British Columbia, Vancouver, BC, Canada
- 24Department of Biology, University of Massachusetts Amherst, Amherst, MA, United States
- 25Department of Biology and Marine Biology, University of North Carolina Wilmington, Wilmington, NC, United States
- 26Department of Biology, Utah State University, Logan, UT, United States
- 27Institute for Biodiversity and Environmental Research, Universiti Brunei Darussalam, Jalan Tungku Link, Brunei
- 28Department of Plant Biology, University of Ilorin, Ilorin, Nigeria
- 29Plant and Soil Sciences Department, University of Delaware, Newark, DE, United States
- 30Universidad de Guadalajara, Centro Universitario de los Valles, Ameca, Mexico
- 31Mechanical, Materials, and Aerospace Engineering, Illinois Tech, Chicago, IL United States
The arboreal ecosystem is vitally important to global and local biogeochemical processes, the maintenance of biodiversity in natural systems, and human health in urban environments. The ability to collect samples, observations, and data to conduct meaningful scientific research is similarly vital. The primary methods and modes of access remain limited and difficult. In an online survey, canopy researchers (n = 219) reported a range of challenges in obtaining adequate samples, including ∼10% who found it impossible to procure what they needed. Currently, these samples are collected using a combination of four primary methods: (1) sampling from the ground; (2) tree climbing; (3) constructing fixed infrastructure; and (4) using mobile aerial platforms, primarily rotorcraft drones. An important distinction between instantaneous and continuous sampling was identified, allowing more targeted engineering and development strategies. The combination of methods for sampling the arboreal ecosystem provides a range of possibilities and opportunities, particularly in the context of the rapid development of robotics and other engineering advances. In this study, we aim to identify the strategies that would provide the benefits to a broad range of scientists, arborists, and professional climbers and facilitate basic discovery and applied management. Priorities for advancing these efforts are (1) to expand participation, both geographically and professionally; (2) to define 2–3 common needs across the community; (3) to form and motivate focal teams of biologists, tree professionals, and engineers in the development of solutions to these needs; and (4) to establish multidisciplinary communication platforms to share information about innovations and opportunities for studying arboreal ecosystems.
Introduction
The arboreal ecosystem is a critical terrestrial environment, fundamental to global carbon, water, and nutrient cycles (Barker and Becker, 1995; Bassow and Bazzaz, 1997; Santiago et al., 2004) and biodiversity maintenance (Ozanne et al., 2003; Nitta et al., 2020). Large trees store a substantial proportion of the world’s terrestrial biomass (e.g., Slik et al., 2013) and forested ecosystems contain nearly half of all global above-ground biomass, primarily in the form of wood in large trunks and limbs (Chen et al., 2014). Remarkably, more water flows through the stems of plants and the pores of leaves than through the mouths of rivers, in the world’s water cycle (Schlesinger and Jasechko, 2014), enough to generate local weather patterns (Makarieva et al., 2014). While the living tissue (cambium, phloem, parenchyma in the sapwood, leaves, buds, and reproductive parts) contributes a small proportion of overall tree biomass, these highly reactive organs represent an immense physiological interface with both the abiotic and biotic environment. Arboreal processes, therefore, have consequences for global biogeochemical, hydrological, and ecological cycles.
Terry Erwin and Janice Scott coined the term “arboreal ecosystem” to capture the vast complexity and importance of the poorly explored above-ground terrestrial environment created by trees (Erwin and Scott, 1980; Erwin, 1982). They identified it as a last frontier for terrestrial biodiversity research. Arboreal ecosystems span the planet and scale in density and structure, from complex multi-layered tropical rainforest to lone urban street trees. The structural complexity of the canopy is a useful proxy for habitat richness and correlates with the biodiversity of dependent taxa (e.g., Gouveia et al., 2014). Structural complexity, in turn, is correlated with canopy height and environmental factors, with the highest complexity found in evergreen tropical rainforests (Ehbrecht et al., 2021) where trees commonly exceed 70 m (Banin et al., 2012; Shenkin et al., 2019).
Arboreal structure influences and is influenced by microclimate, which includes irradiance, temperature, humidity and carbon dioxide concentrations, and is highly dynamic across space and time (Davies-Colley et al., 2000; Newmark, 2001). In temperate zones, bud burst and the initiation of growth after months of winter dormancy occurs over several days, coordinated with local weather patterns. In forests around the world, large synchronous pulses of seed production by plant populations, known as mast events, occur at irregular intervals across years (Kelly and Sork, 2002; Pearse et al., 2020). The many important plant biotic interactions in the arboreal environments include herbivory (Lowman, 2001), pollination (van Dulmen, 2001; Kettle et al., 2011), seed dispersal (Seidler and Plotkin, 2006; Jordano et al., 2007), and seed predation (Janzen, 1971). These interactions can occur on short time scales and early detection can be important for management decisions. Detailed observation and sampling are important for understanding mature tree biology and for identifying critical canopy traits and functions that determine trees’ vulnerabilities to environmentally driven decline (McDowell et al., 2018).
Our ability to understand canopy-dwelling organisms also depends on how we access the arboreal ecosystem, because spatial distribution of canopy-dwelling organisms is inherently three-dimensional. Sedentary and mobile organisms partition themselves vertically according to microclimatic and biotic gradients from the forest floor to the canopy (Petter et al., 2016; Oliveira and Scheffers, 2019), as well as laterally within the canopy from within the trunk to the surface of peripheral branches (Volf et al., 2019). Fine-scale environmental conditions are especially important for sedentary organisms such as epiphytes (Zotz, 2007; Petter et al., 2016) and lichens as well as for organisms with specific niche requirements (Dial et al., 2006). An ability to collect samples in situ and to monitor growth and behavior would greatly contribute to our understanding of the evolution, maintenance, and management of terrestrial biodiversity.
Trees are also immensely important to humans living in cities, as a major component of green infrastructure in the built environment. Trees improve human health (Kardan et al., 2015), help us recover from surgery (Ulrich, 1984), clean the air we breathe (Nowak et al., 2014), and provide numerous other benefits (Turner-Skoff and Cavender, 2019). However, trees in the built environment face several novel challenges. Tree care professionals must monitor tree health and vigor to diagnose pests and diseases and assess the risk of structural failure. The built environment is also capable of harboring a surprising amount of biodiversity and biotic interactions (Trammell et al., 2020) and basic research can help translate the rules of life into cities (Langen et al., 2021).
Little is known about how adult trees balance investments in secondary metabolites against herbivores and resource acquisition. Most study of plant functional traits is limited to seedlings and saplings, which often do not scale to the mature tree (Falster et al., 2018). For example, the abundance and diversity of secondary metabolites appears to be greatest during early developmental phases in plants (Coley and Barone, 1996; Endara et al., 2017; Sedio, 2017). A serious gap exists in our knowledge of how resource acquisition and defensive traits against herbivores and pathogens change across space and time (Barton and Boege, 2017). Collecting leaves for trait mapping, molecular and DNA analyses, species identification and new species discovery remains a challenge, especially in hyperdiverse tropical forest ecosystems. Difficulties of collecting any biotic material from the canopy in such systems delays the advance of our knowledge and development of conservation action to preserve them, as well as the systems they inhabit.
To better understand these arboreal processes, we need more fine-grained data. Canopy research is an active field that supports an international community of researchers (Nadkarni et al., 1996, 2011) but our ability to easily and precisely access the many different dimensions of the arboreal ecosystem remains limited. Even with advanced training and equipment, obtaining the necessary observations and samples with the desired speed and precision presents many practical difficulties. These logistical challenges range from accessing terminal branches and twigs with precision and control to working in remote settings far away from roads, networks, and power sources. To better understand this key habitat, we need new methods that can adequately capture rapid changes in canopy structure, vegetative, and reproductive traits, microhabitat, and microclimate through repeated measurements, across seasons and years. In this paper, we bring together a multidisciplinary, international perspective that includes biologists, tree-care professionals, and engineers to (1) collate existing and proposed access methods for studying the arboreal ecosystem; (2) highlight newly emerging engineering approaches to overcome physical and logistical barriers to accessing tree canopies; and (3) point a way forward to create better modes of access for research and monitoring of the arboreal ecosystem.
The Multidisciplinary Community Seeking Access to the Tree Canopy
To assess the current status of methods for accessing tree canopies, we conducted two online surveys from Oct 23, 2020 to Dec 4, 2020. The first survey, with a total of 27 questions, collected basic information about each respondent’s current ability and future aspirations for accessing the tree canopy (see Supplementary Material 1). Invitations to take the survey were shared through social media platforms, including Twitter, Facebook, and various listservs (e.g., Ecolog, EvolDir, and UrbanForests), and directly with specific groups of interested colleagues, including the now-closed International Canopy Network, staff and collaborators of the Morton Arboretum, and direct messages to specific individuals. In total, 219 people responded, with Ecolog (54), EvolDir (37) and Twitter (26) providing the most completed surveys. Completion of the survey took 7 min on average.
The respondents to the first survey were asked whether they were willing to participate in a subsequent survey and in a continued discussion of how to overcome challenges in tree canopy research. Over three-quarters of the respondents to the first survey (171 or 78%) indicated their interest in participating in the discussion. The second survey was sent only to this smaller group of continuing participants. It contained six open-ended questions designed to gather more detailed information about the specific needs and objectives of the respondents in their tree canopy work (see Supplementary Material 2). We received 51 responses (28% of the short list), and average time to completion was 15 min. Additionally, we held two virtual workshops with the respondents eager to continue their participation in a community discussion. This manuscript and plans for further community development are a result of the virtual discussions, both synchronous and asynchronous, that emerged from the workshops.
Most of the responses (Figure 1) were from researchers who either study trees directly (n = 130 of 216 total; 60%) or study biotic or abiotic elements of the canopy environment (n = 40; 19%), while a smaller fraction manage or care for trees (n = 13; 6%). Respondents with an engineering background were in the minority (n = 7; 5%) with the distribution of the survey biased toward biologists and arborists. Most respondents worked at universities (Figure 2; n = 121; 56%), while non-profits, government agencies, and self-employment were all roughly equally represented (n = 32, 26, 24, respectively). A smaller proportion worked for private companies (n = 13; 6%). Additionally, most respondents were affiliated with institutions in the United States (n = 134 of 209 total; 64%) followed by Germany, Mexico, and France (n = 11, 8, 8, respectively). The trees of interest to the community are found across most land use types (Figure 3); although the greatest fraction is found in natural forests and parks and preserves, a substantial proportion occur on private land, in urban areas, and as part of agroforestry production systems. The trees of interest also span a broad range of vegetation types and seasonal precipitation, with increasing trends toward broadleaved wet forests from temperate to tropical regions (Figure 4).
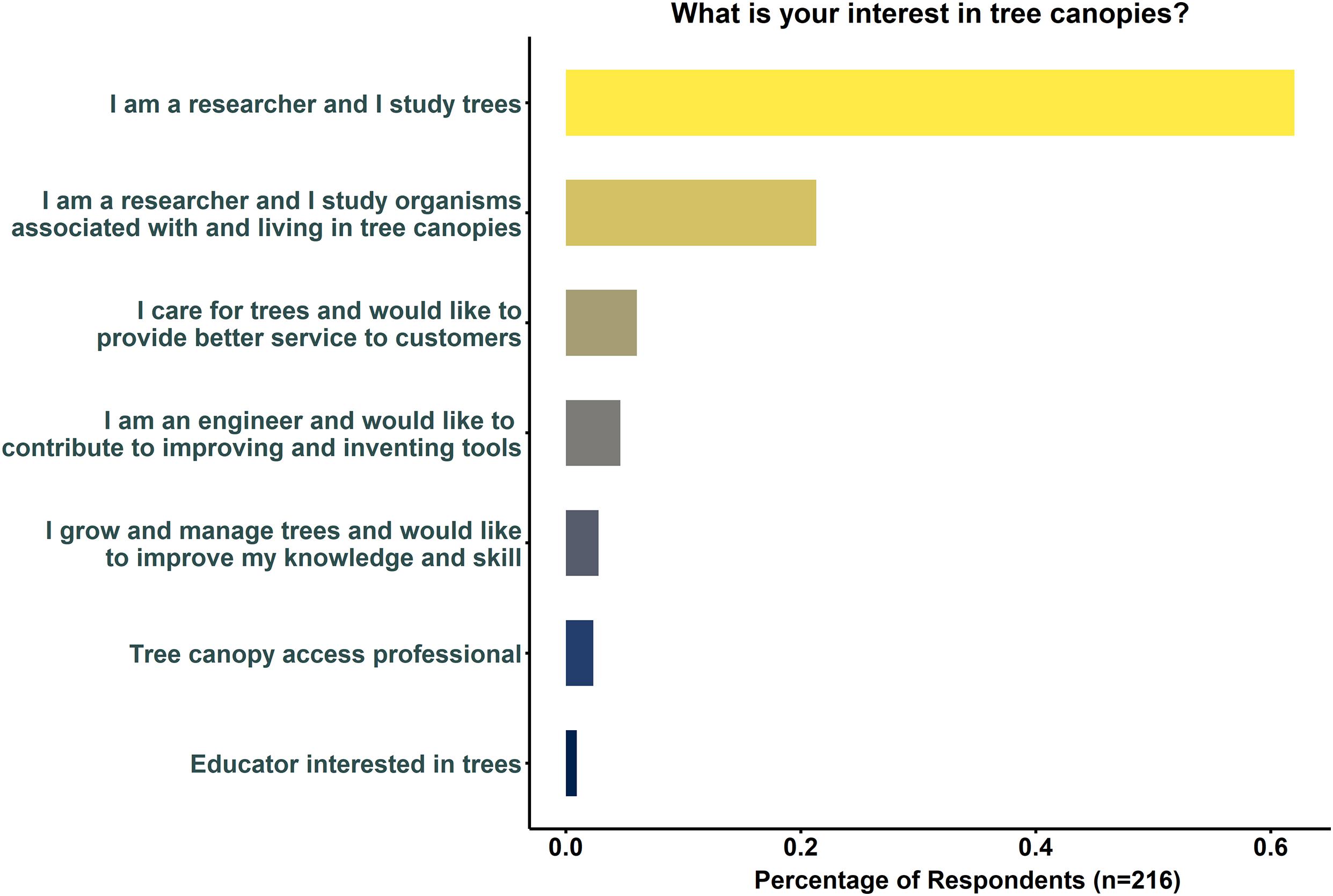
Figure 1. Motivations for gaining access to tree canopies of respondents to an online survey about conducting research and management in the arboreal ecosystem (n = 216). Each respondent could only choose one option. An “other” option was included, which generated the bottom two answers.
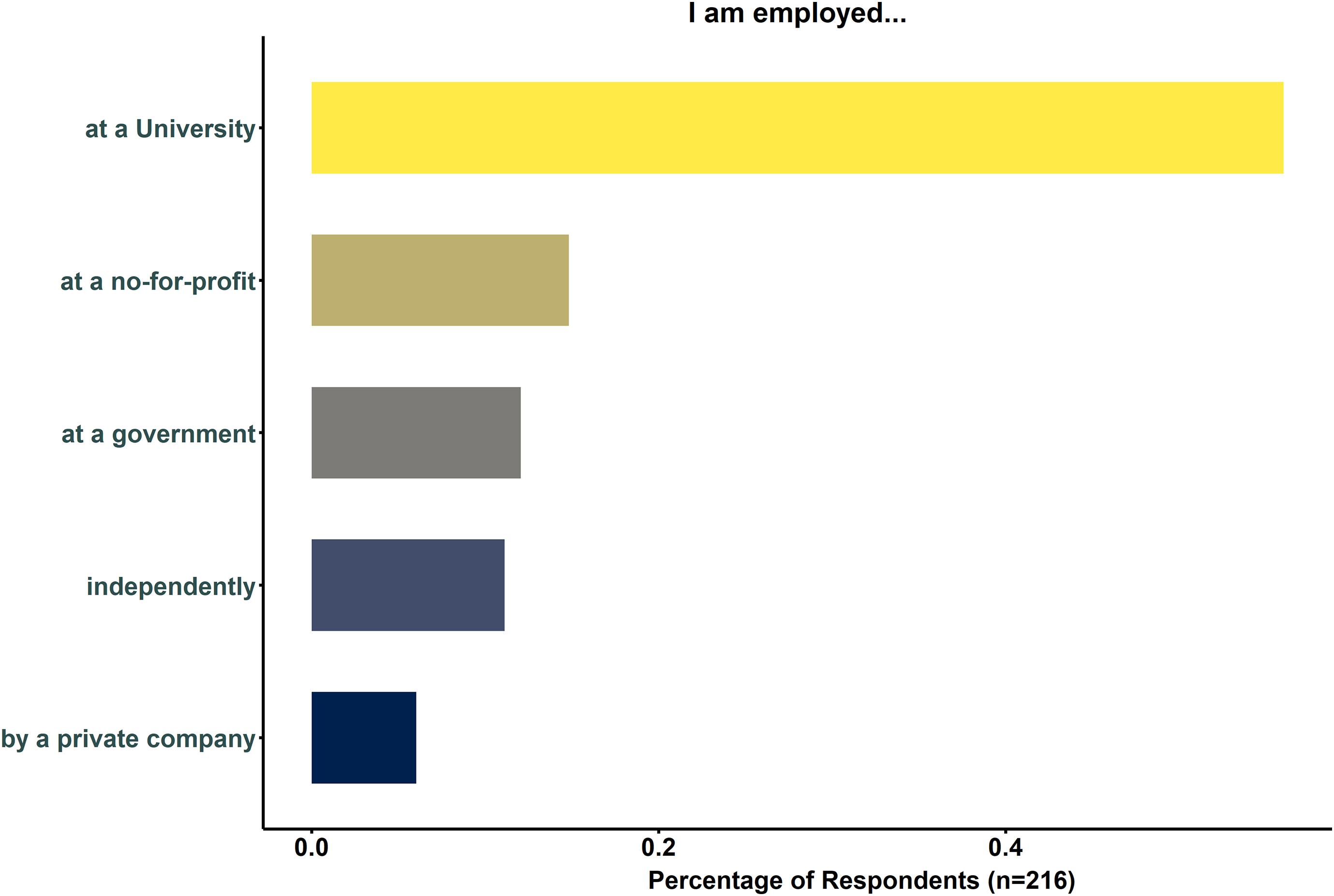
Figure 2. Place of employment of respondents to an online survey about conducting research and management in the arboreal ecosystem (n = 216). Each respondent could only choose one option.
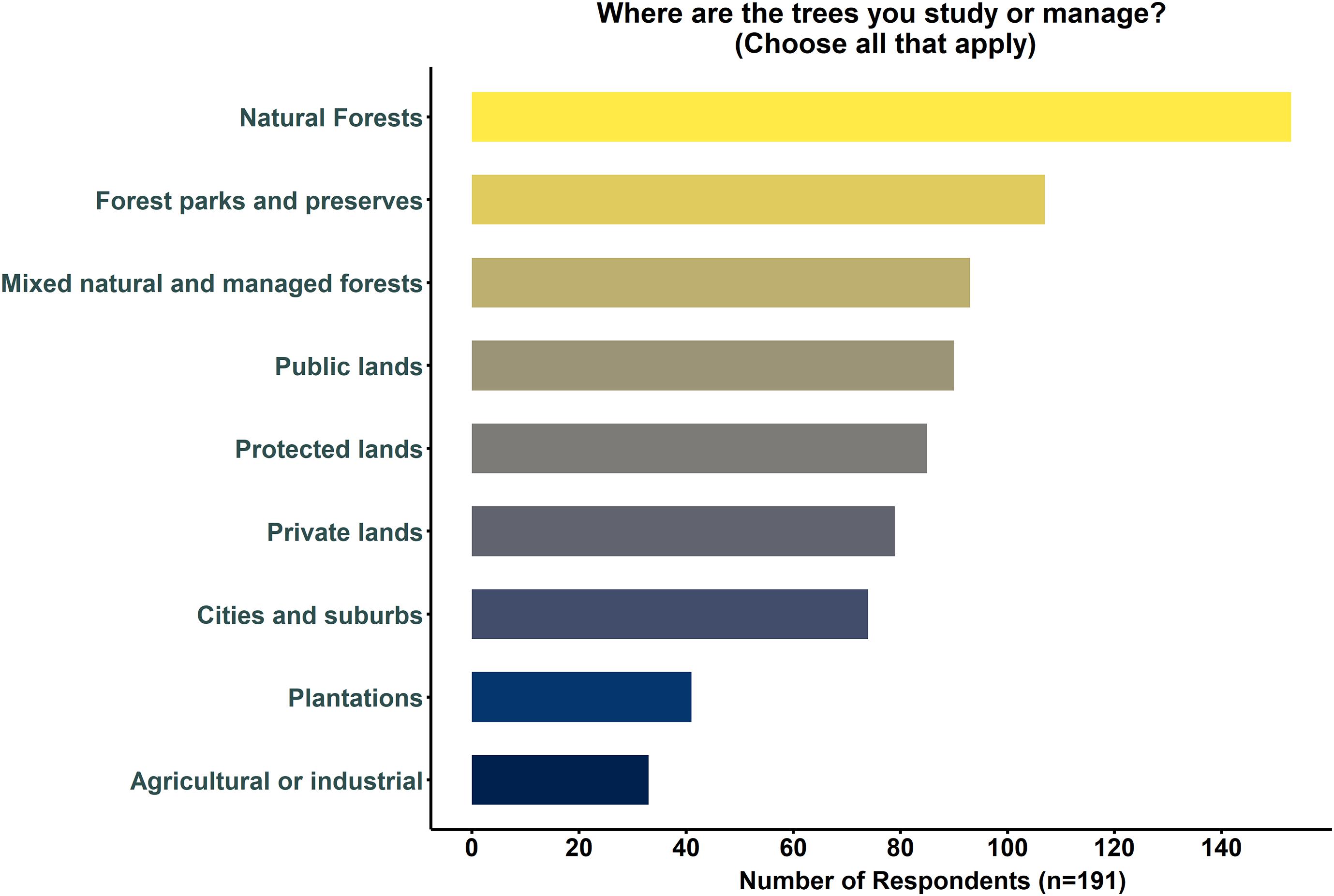
Figure 3. Location of the trees of interest, by land use type, for respondents to an online survey about conducting research and management in the arboreal ecosystem (n = 191). Each respondent could choose all applicable options.
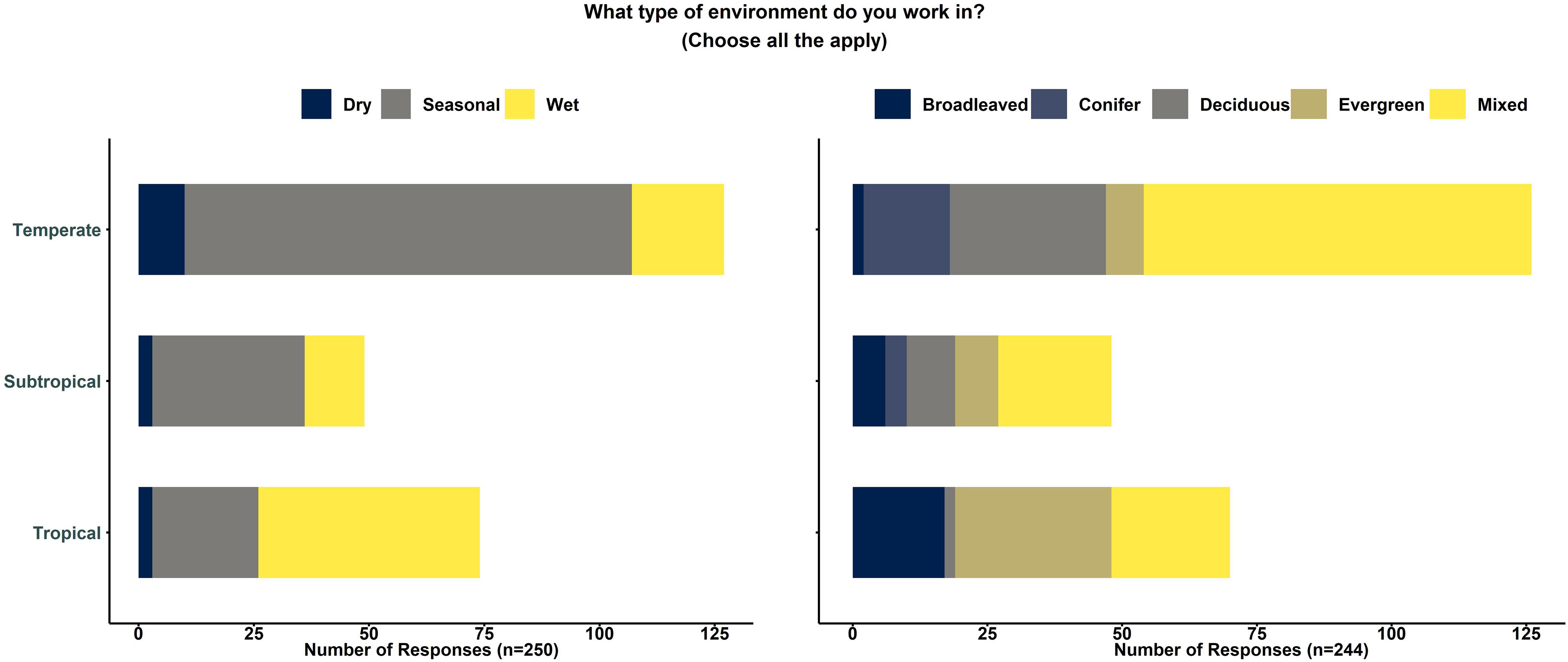
Figure 4. Climate and type of forest where respondents work. Each respondent could choose all applicable options in an online survey about conducting research and management in the arboreal ecosystem.
Overall, the respondents indicated that their access to the tree canopy is constrained (Figure 5). When asked directly whether they felt their access was limited, the median score was 64 out of 100, with higher scores reflecting greater agreement (Figure 5A). Some respondents indicated that they were completely unable to get the samples needed to conduct their research (Figure 5B) and the median was 49 out of 100. Additionally, the tool used to collect the sample often has an impact on which sample was selected (Figure 5C—median of 58 out of 100), further limiting the power of the possible research conducted in the canopy. Finally, there was overwhelming agreement that accessing the canopy took too much time (Figure 5D—median of 66 out of 100) and that time is an important consideration (Figure 5E). The strongest indicator that the researcher requires better access to the tree canopy is apparent when a comparison is made between how often respondents currently access the canopy and how often they would, given an effective and useful tool were available (Figure 6). Clearly, we need better tools to study the tree canopy.
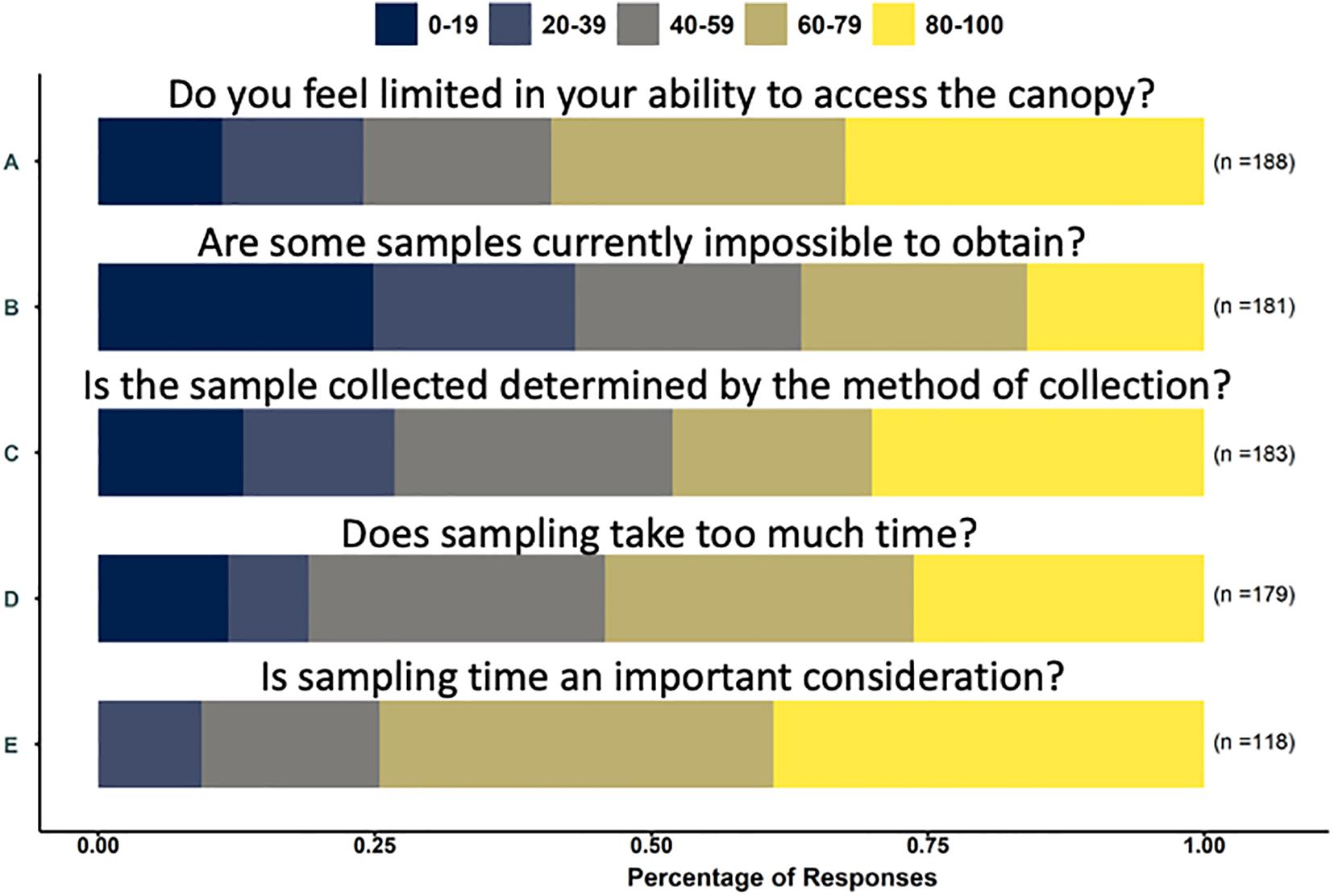
Figure 5. Responses to questions about current ability to access tree canopies from respondents in an online survey about conducting research and management in the arboreal ecosystem. Questions were: (A) Do you feel limited in your ability to access the canopy? (B) Are some samples currently impossible to obtain? (C) Is the sample collected determined by the method of collection? (D) Does sampling take too much time? (E) Is sampling time an important consideration? Respondents expressed their agreement with the question by choosing a number between zero and 100, with 100 being total agreement.
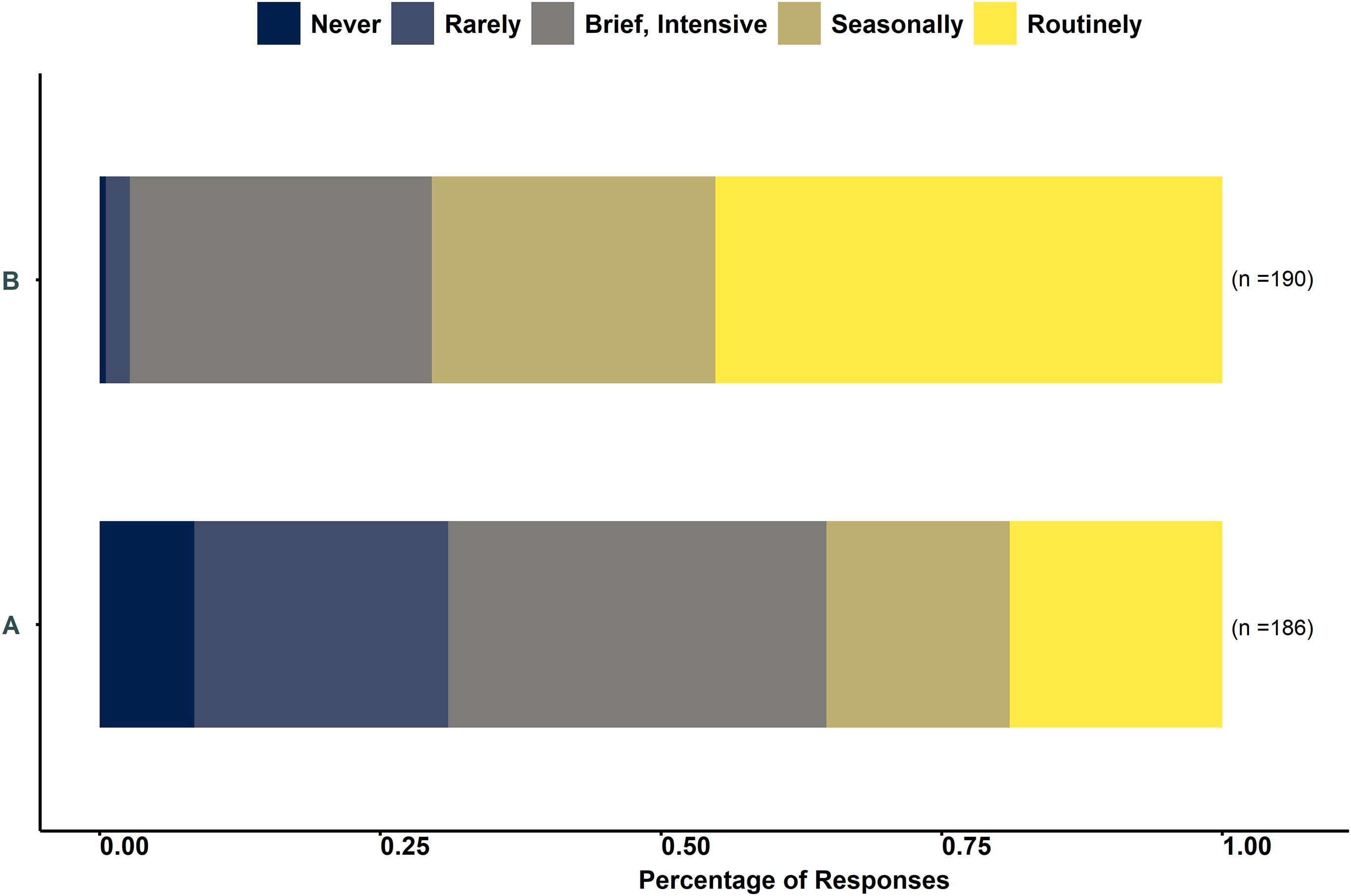
Figure 6. Frequency of tree canopy access reported by respondents given (A) their current methods of access and (B) their desired level of access, if sampling were quick and effective.
Historical and Current Approaches to Canopy Access
The earliest examples of accessing the canopy for research involve the use of local climbers (Wallace, 1869), felling trees (Beccari, 1904), and the construction of elaborate hoist systems (Perry, 1978; Perry and Williams, 1981; Mitchell, 1986). E.J.H. Corner famously trained pig-tailed macaques to collect samples in Malaya (Taylor, 2019) but the approach was impractical and was abandoned (Corner, 2013). Rope-based climbing methods have been a mainstay for canopy access because the equipment is relatively inexpensive and portable (Barker, 2002; Anderson et al., 2015, 2020; Falen Horna and Honorio Coronado, 2018). Cranes, towers, walkways, temporary platforms (Kettle et al., 2011), and even dirigibles have been used to create a stable infrastructure from which people can access the canopy. Researchers gather arboreal samples from the ground by using shotguns, slingshots, throwlines, and fogging entire trees. More recently, Unmanned Aerial Vehicles (UAVs) have created a new avenue to gain a close look and even collect samples from trees. Some of these achievements have been reviewed and celebrated (Mitchell, 1986; Moffett, 1993; Parker et al., 1995; Mitchell et al., 2002; Lowman et al., 2012), stimulating important research initiatives focused on the arboreal ecosystem and the continued improvement of our ability to access the canopy.
In our study, we asked respondents in two online surveys to indicate how they gathered samples from the arboreal environment (in the first survey as multiple choices from an itemized list, including an “other” text option; in the second survey as a free text response). To simplify further discussion, the word “sample” is used interchangeably for the remainder of the article for any type of physical sample, digital image, or measurement data that could be collected. After compiling the results, we categorized these methods for access (Figure 7) based on “how?” and “when?”. How the sample was obtained included: (1) from the ground, (2) climbing the tree, (3) using infrastructure, or (4) piloting an UAV. Sampling frequency included instantly in a single sampling event or continuously over some period of time. Here, we summarize the results of these surveys according to this classification system.
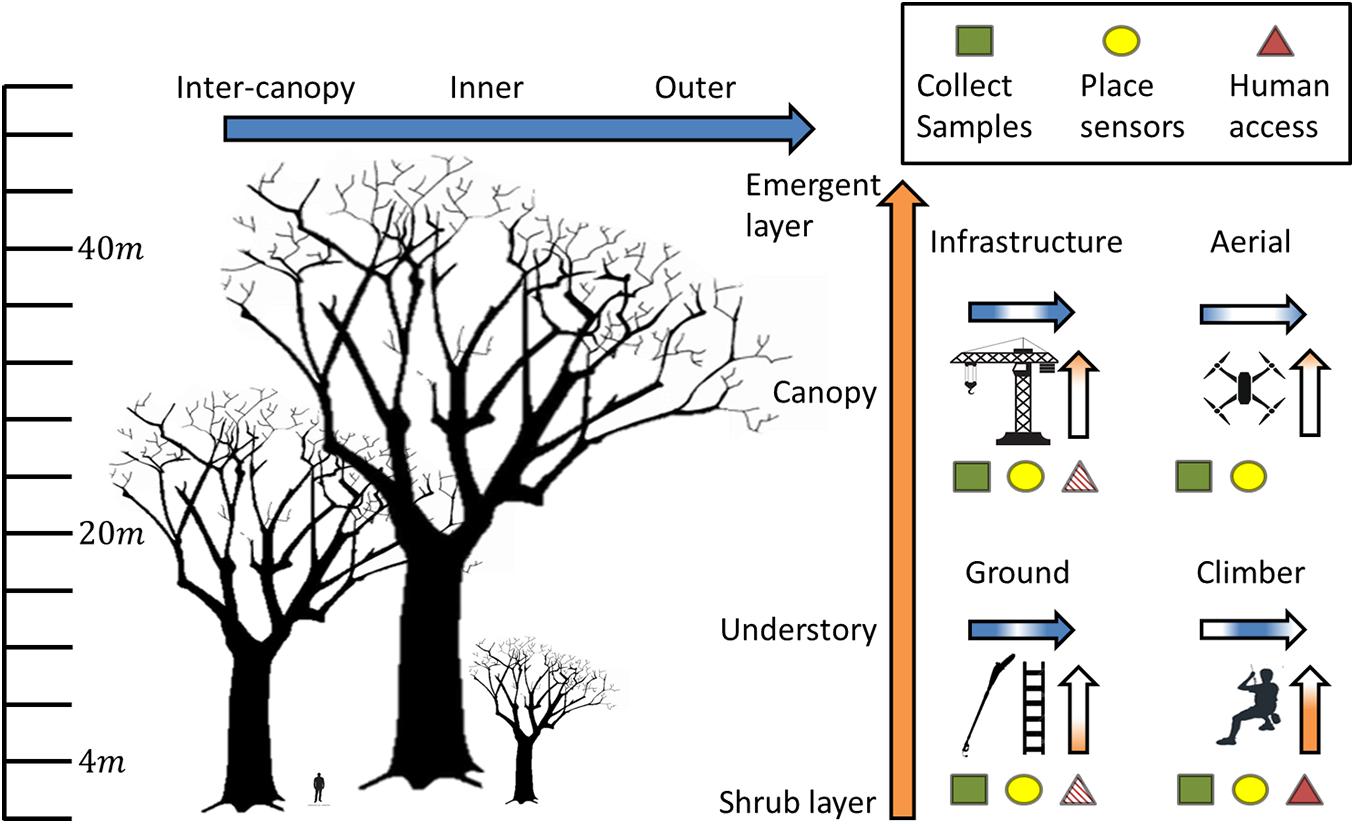
Figure 7. The relative ability of each of the predominant methods of tree canopy access to obtain a sample, given the sample’s position in the tree canopy. The blue shading indicates the horizontal accessibility, from the spaces between tree crowns, the inner crown and the outer crown, left to right. The orange shading indicates the vertical accessibility, from the ground to the emergent portion of the canopy. Shading on horizontal and vertical arrows indicates current access, given each method: darker indicated better access. The modes of sample collection are also shown: instantaneous (collecting a sample) by a square; continuous (place a sensor) by an ellipse; and direct human action by a triangle. Darker shading indicates the overall accessibility by that particular mode.
Our respondents mostly gained canopy access from the ground, with a third (128 out of 384 total answers) indicating that they either used some type of slingshot or shotgun to knock down a sample or they simply searched and picked up samples directly from the ground, which are often dropped by animals as they forage. While this method is fast and easy, it greatly limits the researcher’s ability to objectively choose samples. Generally, imprecision and inability to select the exact location of a sample is a major limitation and this difficulty increases with greater heights and forest structural complexity. Methods requiring a long pole to hoist the sampling device into the tree are limited to relatively low heights in the canopy and necessitate transport of long poles through a forest environment, which is difficult. If upper “sunlit” canopy samples are required, the challenge is even more daunting, and sampling is slow and possibly destructive to the canopy matrix. The use of firearms to shoot down samples also involves a certain amount of personal risk, requires adequate safety training, and is not legal or desirable in all locations, especially in the built environment. The use of ropes to pull down branches can cause significant damage to the canopy and unintended injuries to the tree. Hybrid methods exist by which a line is placed in the canopy (possible using climbing or aerial access) so that sampling equipment can be raised and lowered from the ground. These types of access can also cause unintended structural damage to the tree canopy, which may be problematic for long-term studies.
Climbing was the second most frequently used method (105 out of 384 answers). Rope-based access methods [“rope access”—(Jepson, 2000)] have experienced several innovations over the past two decades. New tools and devices (e.g., hybrid climbing systems that employ both mechanical devices and specialized friction knots) have improved climber efficiency and safety and requiring less physical effort and time. Ropes and specialized equipment for climbing trees are widely available commercially, and relatively affordable and portable, allowing sampling even in remote settings. These methods also involve using slingshots and line launchers to secure the initial ropes and lines. Importantly, with adequate training and expertise, samples can often be obtained from all parts of the tree, including delicate branch tips, epiphytes, and even the open spaces between trees (Anderson et al., 2020), although risk and difficulty increase substantially with taller trees with more expansive, complex crowns.
A main limitation of rope-based methods is that specialized training and experience is needed to execute more sophisticated movements and some trees cannot be safely climbed, for example dead snags growing in isolation from living trees that could permit peripheral access. Many tree-climbing activities are also regulated in most countries with respect to safety in the workplace, as this activity exposes operators to the risk of falling from height (Longo et al., 2013). Climbing can also be time consuming and tiring, so ultimately the number of trees climbed, and number of samples gathered within a time frame is constrained. Sampling can also only be performed on structurally sound trees and repeated rope access must be carefully planned to minimize damage (Chavana-Bryant et al., 2019). Finally, rope access can be combined with other methods, allowing vertical and horizontal movement away from cranes, walkways, and towers (see below). When sampling is performed by a human, rope access offers a flexible and effective means for unbiased and replicable sampling in tree canopies.
Construction cranes (Nakamura et al., 2017) and canopy walkways or towers (Lowman and Bouricius, 1995) require the greatest initial investment, but once installed require no further training or specialized equipment other than the assistance of a crane operator. Therefore, they provide access across the widest range of people, considering age and physical ability. Further, they allow ready access to the open spaces between trees. The physical infrastructure of cranes and walkways cannot reach the interior tree crown, however, limiting access to the outer crown and branch tips without some type of augmentation or sampling device. They also only provide access to a fixed limited number of trees and environments in the immediate vicinity of the crane or walkway. Additionally, sampling from this population of trees must be carefully managed to avoid disturbing or changing the environment, which can affect long-term study results. because moving platforms through the canopy can cause damage if not done carefully. Other types of infrastructure include bucket trucks and mechanical means of lifting a person into the tree canopy. These tools provide excellent access to the outer canopy but require substantial capital investment and are only applicable when the tree is accessible by road and grows in the open.
Several of the respondents (50 out of 384 answers) use aerial techniques to gain access to the canopy. A small proportion reported use of mobile infrastructure, such as flying balloons or rafts (Hallé et al., 2000; Mitchell et al., 2002; Dorrington, 2005) but these remain expensive, logistically difficult to transport, and unavailable to most scientists. Remote sensing with drones was the most common aerial method used to sample the canopy. Over the past few years, Unmanned Aerial Vehicles (UAVs) have evolved rapidly and been adopted by scientists in a multitude of applications. Declining acquisition costs, increasing reliability, and ease of use have driven demand, while airspace regulators have enacted rules to facilitate low-risk UAV operations. Collecting physical samples with a UAV has also become possible over the past few years. The first devices used cutting blades or knives attached firmly to the UAV through a pole.1,2 This, however, can create vibrations and destabilizes the UAV when it meets a branch. A critical development was the suspension of the sampling device beneath the drone. This configuration allows stable operations and a greater sampling reach while keeping the UAV at a safe distance from natural obstacles.
New aerial sampling tools were developed by various labs with multiple actuators and a vision system to assist the operator during sample collection (Finžgar et al., 2016; Käslin et al., 2018; Kutia, 2019; Charron et al., 2020). Among the solutions available on the market, the DeLeaves canopy sampling tool (Charron et al., 2020) has now been used by scientists in a number of field experiments (NEON, 2019; Schweiger et al., 2020; Arseneau et al., 2021). This tool allows tree canopy samples to be collected in about 6 min (i.e., from UAV takeoff to return with the sample) and has proven to be effective in a variety of environments and for more than 30 tree species. The approach also removes a substantial amount of direct human risk involved in other methods, when people are climbing, shooting, or being suspended to collect samples. Recent work used the DeLeaves canopy sampling tool on a custom UAV to assist the pilot during sampling. In that system, an RGB-D camera was used to detect and locate suitable branches for sampling before an on-board computer guided the UAV to autonomously collect the selected branches (La Vigne et al., 2021). This assistance significantly reduces the pilot workload and accelerates the sampling process by more than six times.
UAV sampling still has limitations. For example, it is necessary to maintain a safe distance from the top of the tree to avoid any contact with the propellers. This limits sampling to the upper third and the outer edge of a tree. Finally, while battery life has improved, flight time and payload remain limited (e.g., the DJI M300 has a 9 kg max takeoff weight, a 2.7 kg max payload, a 55 min max flight time and a max speed of 23 m/s). However, in practice, the flight time when fully loaded with sensors and sampling equipment is closer to 15 min. For missions of long duration, multiple sets of batteries need to be carried and recharged in the field when possible (e.g., the DJI M300 has a 2.7 kg battery pack of 548 Wh). The noise associated with UAVs may also disrupt organisms inhabiting natural ecosystems. While there are instances of UAVs having been attacked by birds (Yaacoub et al., 2020; Chaari and Al-Maadeed, 2021), which can disrupt research progress and harm birds, best practices have been developed to fly UAVs around birds (Junda et al., 2015). Noise or activity produced may also create other types of unsafe situations for researchers. For instance, the UAV noise may disturb unseen nests of bees or wasps, which in one instance has resulted in the death of a researcher due to bee stings (pers. obs.).
The respondents also pointed out that the site context can also present significant challenges for gaining canopy access. In remote locations, beyond the simple fact that newer technologies require electrical power, complicating their heavy implementation, their noisy operation and high visibility can call attention to researchers working in areas where illegal activities or military conflict are occurring. Developed and populated landscapes, such as cities and towns, present different challenges associated with tree canopy access. While line launching techniques (e.g., slingshot) are used in natural forested areas within cities, they pose considerably more risk in urban forest settings used for recreation, endangering bystanders and property. UAV-based techniques pose additional challenges associated with permission and security concerns in populated landscapes. Finally, sensors or other types of equipment placed within urban tree and forest canopies for continuous or repeated measurements can be stolen or damaged.
Modes of Access and Experimental Design
Beyond the practical details of how the sample is collected, when and where the sample is collected is potentially of even greater importance. Previously, canopy sampling has tended to be arbitrary, non-random, unrepresentative, and opportunistic. Also, low replication or pseudoreplication (sensu Hurlbert, 1984) are potential weaknesses. Yet few canopy-based studies report sampling issues (Kapos et al., 1993; Baldocchi and Collineau, 1994; Koch et al., 1994), although logistics and experimental design in arboreal ecosystem research remain undeniably challenging. But the focus among researchers has shifted from simple access to experimental design and sampling issues (Barker and Pinard, 2001; Bongers, 2001; Nakamura et al., 2017) centered on hypothesis driven quantitative approaches (Rinker and Jarzen, 2004; Volf et al., 2019). Ideally, modes of access and experimental design of arboreal ecosystem research need to be equally rigorous as other terrestrial ecosystem research (Barker and Pinard, 2001; Mitchell, 2001; Sutton, 2001). The quantity and quality of data and samples collected in the canopy should not be compromised based upon how and when the sample can be collected. For example, researchers are not always sufficiently mobile in the upper canopy, so to avoid under- or over-sampling or pseudo-replication, independent replicates can be achieved spatially, by simultaneous sampling by researchers, sensors or traps (e.g., Bassow and Bazzaz, 1997; Bar-Ness et al., 2012) or temporally across days or weeks (e.g., Perez-Salicrup et al., 2001). Devising the best strategies for achieving these comprehensive approaches requires collaboration and coordination across the community of arboreal researchers.
Modes of access and experimental design can vary considerably depending on the aspect of the arboreal ecosystem that is being sampled. For example, the epiphyte community requires capturing the plants living attached to the limbs and branches of a tree. The limited access to the canopy has been calculated to miss, for example, more than 50% of lichen diversity (Boch et al., 2013), much of which is endemic to the upper canopy (Fritz, 2008; Ellis, 2012; Marmor et al., 2013). To study epiphyte communities and obtain complete species lists during inventories, various complementary methods are used, including surveying fallen twigs and branches (Sarmento Cabral et al., 2015; Gasparyan et al., 2018), using tree pruners (Degtjarenko et al., 2016, 2020), and employing rope-based methods (Fanning et al., 2007; Boch et al., 2013; Kiebacher et al., 2016). Canopy crane access has permitted detailed characterization of epiphyte assemblages across forest strata, but these have been limited to only a few locations (Zotz, 2007; Petter et al., 2016) and gondolas can damage the upper or outer canopy. Trees or branches felled for other purposes or that naturally fall also permit comprehensive sampling of epiphyte diversity (Marmor et al., 2013; Kaufmann et al., 2019). The latter methods are not always applicable in regular surveys or permissible in highly protected forests.
The collection of arthropods and other invertebrates from tree crowns relies on a multitude of sampling methods, including fogging from the ground and insect traps (e.g., Malaise, flight interception, pitfall, sticky traps) that can be lifted with ropes into trees. The integrative IBISCA (Investigating the Biodiversity of Soil and Canopy Arthropods) approach is a good example of the combination of these methods with a wide variety of canopy access tools (e.g., cranes, balloons, rafts, climbers) leading to the development of new sampling protocols (Leponce et al., 2012, 2021). Recent large-scale, multi-taxa projects to access the tree canopy have relied on tree felling, canopy cranes, cherry pickers (Nakamura et al., 2017; Volf et al., 2019; McCaig et al., 2020; Mottl et al., 2020), or ground-level fogging using insecticide (Swart et al., 2020). Canopy-dwelling vertebrates including birds, frogs, lizards, and mammals are commonly studied observationally either using binoculars, climbing into the canopy, or using remotely sensed images to identify nests (Milne et al., 2021). For more sentient taxa (birds and mammals) and for behavioral studies in particular, there is risk of unintended disturbance or demonic intrusion (Hurlbert, 1984) when researchers introduce themselves into the arboreal environment, thus changing the naturally occurring parameters in the experiment (Barker and Pinard, 2001). Censusing from the canopy accurately captures richness patterns (Anderson, 2009) and can elucidate patterns of vertical stratification (Scheffers et al., 2013). Traps to capture small mammals have been implemented in the canopy and can be installed directly or from the ground (McClearn et al., 1994; Kays, 1999; Lambert et al., 2005).
While methods of access are generally the focus of experimental design, an important distinction about the timing of access was identified in remote discussions among the co-authors, during and after the virtual workshops. Specifically, samples are either collected (1) instantaneously or (2) continuously. Instantaneous samples serve as a snapshot in time, typically involving a physical sample, a measurement, or an image or data taken by cameras or sensors, that is collected a single time without expectations of returning to the site. Continuous samples, on the other hand, can involve either placing a sensor or trap in the tree or repeated measurements of the same part of the tree. These two approaches require fundamentally different techniques and impose different functional constraints on the experimental design. Most methods used by canopy researchers focus on instantaneous sampling, where a physical specimen is removed from the tree. Continuous monitoring and repeated measurements are undergoing a revolution because of advances in sensor technology and camera traps. Sensors have been placed in tree canopies using UAVs (Farinha et al., 2020) and improving upon these methods is one major avenue for innovation. Additionally, repeated sampling of the same tree, preferably even the same part of the canopy, can be necessary during experimental trials or long-term monitoring programs. These repeated measurements often involve the construction of canopy infrastructure but UAVs again might provide a new means of achieving this research. Controlling the structural and physiological impact and logistical burden of these repeated sampling techniques is important aspect to consider (Zandt, 1994).
Remote sensing provides information about forest canopies through a sensor signal resulting from the interaction of electromagnetic energy with the canopy components (Weishampel et al., 1996; Baret and Buis, 2008). The level of detail and type of information obtained from the canopy mostly depends on the type of platform (satellite, airborne, UAV) and sensor (multi/hyperspectral, radar, laser) used. Traditional remote sensing uses satellite imagery and it has improved in terms of the type of sensors, spatial and temporal resolution (Chavana-Bryant et al., 2019; Lechner et al., 2020). UAVs provide relevant canopy information at flexible times, with a higher spatial resolution and a relatively cheaper price when compared to satellite data (Lechner et al., 2020; Dainelli et al., 2021). Arboreal camera traps are effective at capturing photos of canopy vertebrates if foliage is removed from the immediate vicinity of the camera to prevent false triggering (Di Cerbo and Biancardi, 2013; Gregory et al., 2014; Whitworth et al., 2016; Bowler et al., 2017; Nazir and Kaleem, 2021). Fixed cameras in the canopy as Phenocams or camera traps also provide valuable temporal information to study growth, phenology, harvest traits (Aasen et al., 2020). Ecoacoustic monitoring is an emerging field (Ducrettet et al., 2020; Pérez-Granados and Traba, 2021) that could be adapted to canopy research similarly as cameras.
The combination of machine learning techniques with remote sensing data allows several canopy studies ranging from semi and automatic identification and quantification of canopy species using conventional RGB cameras (Tagle Casapia et al., 2019; Ferreira et al., 2020; Wang et al., 2021), multispectral cameras (Gini et al., 2014; Wagner et al., 2020) or hyperspectral sensors (Dalponte et al., 2014), to assess health status (Dainelli et al., 2021), phenology (Feng et al., 2021), above-ground biomass estimation/quantification using RGB, radar or Lidar (Marks et al., 2014; Brede et al., 2019; Dainelli et al., 2021), canopy traits (Thomson et al., 2018; Ganivet and Bloomberg, 2019), or to detect fauna with thermal sensors (Spaan et al., 2019; Zhang et al., 2020). In all the cases, to be most effective, calibration against carefully chosen samples at the top of the canopy is required to provide accurate results (Käslin et al., 2018; Chavana-Bryant et al., 2019; Schweiger et al., 2020). In addition, while we are aware that some high-tech methodologies such as airborne laser-guided imaging spectroscopy could help to solve these issues especially in tropical forests, these methodologies are expensive and not accessible for all researchers (Baraloto et al., 2010; Asner et al., 2017). Thus, setting a workflow that allows drone-based leaf sampling and drone-guided imaging spectroscopy would be valuable in terms of cost reduction and canopy data accessibility.
Engineering and Robotic Possibilities
Given the need for more precise and versatile ways to access the arboreal ecosystem, both for instantaneous sampling and for continuous monitoring, recent progress in robotics can enable new capabilities. Historically, robotics has been used to perform tasks known as “the 3Ds” (typically, dull, dangerous, or dirty, but also sometimes difficult, demeaning, and demanding), particularly repetitive tasks in manufacturing and warehouse fulfillment (Bogue, 2016). Sensors, sometimes located on mobile robots to create deployable and reconfigurable sensor arrays, are also used to monitor certain areas for extended periods of time and in a variety of environmental conditions. Given these examples, one can presume that teleoperated robots could be deployed in the exploration of a tree canopy during an expedition, to address many of the sampling issues discussed above. Automating data collection can also provide solutions to creating large, replicable datasets and collecting repeated measurements. To inspire new ways to perform canopy research, we provide a short review of recent relevant robotics progress and the challenges that remain.
Robots are typically classified either as manipulator arms or mobile robots. Manipulator arms are common in factory settings, moving objects or performing tasks such as painting or welding. While manipulators may employ different joints and linkages, they are generally similar to each other and could best be deployed along with canopy infrastructure, where a fixed anchor point would be available. For example, when placed on a canopy crane, a manipulator arm could extend the reach and precision of sample collection. Mobile robots have a much more varied form and are typically classified according to their mode of locomotion. These include wheeled, tracked, and legged (or combinations thereof) robots for terrestrial locomotion, and fixed-wing, rotorcraft, or ornithopters (flapping wing) systems for aerial flight.
Many of the challenges of mobile robot mechanical design lie in understanding how to move in challenging environments, including deformable terrain such as sand or mud, overcoming specific obstacles (like stairs), or climbing vertical surfaces. Tree-climbing robots have been developed, using wheels (Megalingam et al., 2021), arrays of small toes with sharp spines that engage with asperities in the climbing substrate, also known as microspines (Megalingam et al., 2021), “legs” that grasp the trunk (Lam and Xu, 2011), or trunk jamming mechanisms.3 These platforms are much slower than human climbers and none have demonstrated the ability to reliably and repeatedly navigate trunks with branches. Other robots can deploy observation masts up to 12 m high using three interlocked steel tapes4 or grow like a vine by everting thin plastic tubes using compress air (Haggerty et al., 2021). These could be deployed from the ground, from within the tree canopy, or from aerial platforms; however, they have many logistical issues that limit their successful deployment for research.
Aerial platforms have the advantage of being able to rapidly fly over an area to easily access the topmost branches of the tree canopy or for reconnaissance to identify and target sampling sites. In general, above-canopy flight is a simple task because of the presence of Global Navigation Satellite System (GNSS) signals, such as GPS, which allow the robot to accurately localize itself in the environment. However, below-canopy flight is much more difficult because the canopy effectively blocks all GNSS signals. Thus, aerial platforms that navigate below the canopy must be teleoperated or rely on sophisticated sensors such as vison or lidars coupled with advanced control and path planning algorithms for the robot to adequately localize itself. When operating above the canopy, suspended payloads could be transported by these platforms to collect samples or retrieve sensors near any structure in the canopy. UAVs have also been flown with nets to capture insects (Löcken et al., 2020) or various devices to capture spores (Crazzolara et al., 2019).
Although direct contact with arboreal elements (leaves, twigs, fine vines) should generally be avoided, some UAV platforms are protected against intermittent contact (e.g., through physical guards or duct around propellers) or are even designed to take advantage of direct contact with their environment (e.g., using cages around the drone (Klaptocz et al., 2013; Kalantari and Spenko, 2014). This allows UAVs to fly within forests and inspect enclosed spaces, crevasses within glaciers, tunnels, or underground mines. Some aerial platforms can also land on high power electrical lines for in-contact inspection (Mirallès et al., 2018) or perch on surfaces/branches of various orientations for extended monitoring (Roderick et al., 2017; Hang et al., 2019; Mehanovic et al., 2019; Nguyen et al., 2019). Many new commercial UAVs now also have sensors to autonomously avoid obstacles when navigating around hiking trails, construction sites, or underground mines (e.g., Skydio, Emesent).
Mobile robots are commonly used to carry sensors to measure values of interest. The sensors (e.g., RGB cameras, range sensors such as lidars or radars, Infrared (IR) sensors, and hyperspectral cameras, among others) transported by the robot could be used to reconstruct the 3D environment through photogrammetry or point clouds (Scher et al., 2019). Many robots are also equipped with manipulator arms including end-effectors to interact with the environment (Zhang et al., 2020) through grasping or specialized samplers (e.g., branch cutter, aspirating devices, pumps, drills) to collect branches, flowers, bark, water, or insects. Robots could also be used to precisely install ropes or as a platform to deploy instrumented darts (Farinha et al., 2020) or other projectiles. Many industrial fields are investing heavily to advance robotics technology. These include aerial manipulation for structural inspection and maintenance, agricultural robotics, mining, manufacturing, fulfillment centers, medical robotics, autonomous driving, among others. Many of the technologies in these fields could be re-used to provide better access to arboreal ecosystems for research and management.
One of the main challenges with robotics is the required resources, expertise, cost and time needed to develop, use, and maintain these systems. To successfully develop tools that will be used in the field, coordinated efforts between scientists and engineers are required. Priority should be established by identifying the most pressing and common needs from scientists, but also the needs for which viable technological solutions can be developed by the engineering team. Equipment sharing should be favored, as well as concerted sampling efforts (i.e., collecting samples that can provide data for multiple studies). Technological solutions that can be used in multiple contexts can also be beneficial. For example, the creation of robotic augmentation to extend and improve the human ability to sample specific parts of the tree in structurally challenging regions of the canopy could be useful either for someone working from the ground or for someone who has climbed up the trunk of the tree. Likewise, devices developed to allow a hovering UAV to place a sensor precisely in the canopy could also be used by a person working from a canopy crane or walkway.
Future Goals and Directions
Tree canopy research has grown substantially over the last three decades (Moffett and Lowman, 1995; Barker, 2015; Lowman, 2021), with an acceleration in innovative techniques and possibilities led by technological developments in sensors, robotics, and UAVs. Here, we hope to facilitate this acceleration and focus innovation on the tools and technologies that could have the broadest impact and encompass the entire arboreal ecosystem. Experimental design, scientific questions, and management practices should not be determined by the methods and modes of accessing tree canopies. The community of scientists who study the biology of tree canopies originally had to overcome tremendous obstacles and recent developments are both a cause and a consequence of creative methods for accessing tree canopies (Barker and Sutton, 1997). Even today, most people working with trees state that their canopy access is limited and if they had effective and accurate equipment, they would routinely collect samples from the arboreal ecosystem.
This study represents a first step toward building a multidisciplinary community of academic scientists, tree professionals, and research engineers to address the limitations we face in accessing the arboreal ecosystem. The results provide important information from diverse perspectives about current abilities, limitations, and aspirations. Our virtual discussions, both synchronous and asynchronous, have created an effective framework for characterizing the methods and modes of accessing the canopy. To find common ground among these many interests and to maximize the impact for global efforts to study the arboreal ecosystem, we have identified four main priorities to advance these efforts:
1) broaden participation geographically and professionally;
2) identify the most common needs;
3) create teams of those who need access, e.g., biologists, with those who can help provide access, e.g., engineers and climbers, organized around common needs; and
4) establish a communication platform for sharing information.
Broadening participation, both geographically and professionally, will allow an effective interchange of advances, an efficient communication channel about different end user’s values, and create opportunities for novel insights from under-represented perspectives. During the initial stages of our discussions, the responses to our survey were dominated by those working at institutions and universities in the United States and the United Kingdom. Subsequent discussions, during the virtual workshops and asynchronous collaborations on this manuscript, were more diverse, as demonstrated by the authors here. A more focused effort to include participants from tropical countries, is needed (Botanic Gardens Conservation International [BGCI], 2021). In some of these countries, the resources and infrastructure for research can be limited (e.g., Jarrín-V et al., 2021) or the value and emphasis of different types of research can be substantially different (Ciocca and Delgado, 2017).
Tree managers and tree care professionals, both municipal and private, work with trees every day and have a great deal of experience and motivation to access tree canopies. The value of trees in our built environment, both for human health and pleasure, has become increasingly apparent to the general public and city planners (Matsler et al., 2021). Combating pests, treating diseases, pruning, and assessing structural integrity of trees would all be aided by easy, precise, and low-cost access to the tree canopy. Cheap and enjoyable means of obtaining arboreal samples could also allow community scientists to meaningfully participate and contribute to research related to community health, environmental justice, and important scientific questions about urban ecology and evolution (Langen et al., 2021). This connection between the public and the arboreal ecosystem can also be a powerful way to educate and inform students and tourists about its importance and value, even generating forest conservation and sustainable alternative incomes for indigenous people. To engage participants from these two different segments of global society, we will contact relevant societies and associations, international and national, like the International Society for Arboriculture, to learn how to make their members aware of our activities and objectives.
By identifying the common needs, we will form working groups that connect the scientists and professionals who need routine and efficient access with the engineers and roboticists with the appropriate expertise and interest to improve and invent the needed tools. Combining technical expertise from these two scientific fields may provide solutions through the application of existing technologies to the challenges faced in gaining canopy access (Cannon, 2012). By starting with the “lowest hanging fruit,” we can have the broadest impact and understand the functional requirements and engineering approaches that span several modes of access. The intersection of the means of access (e.g., canopy infrastructure, climbing, UAVs) with the needs of access (e.g., physical leaf sample, multispectral imagery, arthropod samples) will determine the best strategy for designing and improving these tools and how we might best incorporate robotic and engineering solutions.
Effective communication is important to any distributed and multidisciplinary effort. Given the rapid pace of innovation and change in global circumstances, we seek to establish an effective platform, built on existing social media networks and digital sharing technologies. This communication would not only coordinate activities and discussions within the network but would act as an information hub for technological advances, training opportunities, community events, and even expeditions. Informing the community about canopy samples being collected for one purpose can potentially have significant and simultaneous value for multiple research programs, especially for studies of sedentary plant associates (e.g., fungi, lichens, bacteria, mites, scale insects, whiteflies) and for samples collected in poorly known and remote tropical forests and other biodiversity hotspots. Researchers would benefit from standardized protocols for sample and data collection and distribution to allow for comparative studies across taxa and projects. The organization of workshops at large scientific and professional conferences, targeted events with municipalities and non-profits, and continued online virtual engagement will be an important part of growing the community and increasing inter-disciplinary and cross-agency collaborations and networking.
Finally, we recognize that expense and training will remain a barrier to entry for many people whose professional or personal needs do not require regular tree canopy access. Efforts to remove these barriers, to maximize benefits and share the load as much as possible, will be sought. For example, the creation of regional hubs of expertise and equipment to facilitate the use or even provide service of canopy sampling tools or personnel to occasional or one-time users in the scientific and public communities, potentially even on a contract basis. This would help the community combine efforts and maximize benefit obtained from existing tools and expertise and innovate on new ones. Moreover, an awareness of the types of specimens sought by other researchers could potentially provide an easy solution for obtaining samples simultaneously for several purposes.
Data Availability Statement
The raw data supporting the conclusions of this article will be made available by the authors, without undue reservation.
Ethics Statement
The studies involving human participants were reviewed and approved by the Illinois Institute of Technology. Written informed consent for participation was not required for this study in accordance with the national legislation and the institutional requirements.
Author Contributions
CC, CB, and MS conceived of the project and conducted the surveys. CC, CB, MS, and AD organized and led the workshops. CC and CB analyzed the data. CC, CB, DA, GA, MB, GC, and JR made substantial creative contributions to the manuscript. All authors contributed to the writing, editing, and revising of the manuscript.
Funding
The research presented in this article was supported by the Center for Tree Science at The Morton Arboretum.
Conflict of Interest
Some of the authors provide professional services, like tree climbing or sell UAV sample collection devices, that are discussed in the paper. The lead author and most of the other authors have no conflict of interest and have paid careful attention to any possible advocacy or promotion of a particular service caused by these select few potential conflicts.
Publisher’s Note
All claims expressed in this article are solely those of the authors and do not necessarily represent those of their affiliated organizations, or those of the publisher, the editors and the reviewers. Any product that may be evaluated in this article, or claim that may be made by its manufacturer, is not guaranteed or endorsed by the publisher.
Supplementary Material
The Supplementary Material for this article can be found online at: https://www.frontiersin.org/articles/10.3389/ffgc.2021.712165/full#supplementary-material
Footnotes
- ^ “How Mythbuster Jamie Hyneman Hacked a Drone to Trim His Trees.” Popular Mechanics, Popular Mechanics, 14 Nov. 2017, www.popularmechanics.com/flight/drones/a26102/jamie-hyneman-drone-plants/.
- ^ “Sampler Drones for Forestry Research.” Rausser College of Natural Resources, UC Berkeley, nature.berkeley.edu/garbelottowp/?p=1801.
- ^ https://www.youtube.com/watch?v=2qKDixMGaqY&ab_channel=KAZ-corpLTD
- ^ https://www.faulhaber.com/en/markets/factory-automation-robotics/zippermast/
References
Aasen, H., Kirchgessner, N., Walter, A., and Liebisch, F. (2020). PhenoCams for field phenotyping: using very high temporal resolution digital repeated photography to investigate interactions of growth, phenology, and harvest traits. Front. Plant Sci. 11:593. doi: 10.3389/fpls.2020.00593
Anderson, D. L. (2009). Ground versus canopy methods for the study of birds in tropical forest canopies: implications for ecology and conservation. Condor 111, 226–237.
Anderson, D. L., Koomjian, W., French, B., Altenhoff, S., and Luce, J. (2015). Review of rope-based access methods for the forest canopy: safe and unsafe practices in published information sources and a summary of current methods. Methods Ecol. Evol. 6, 865–872. doi: 10.1111/2041-210X.12393
Anderson, D. L., Schulwitz, S., May, M., Hill, G., Koomjian, W., and McClure, C. (2020). Can increased training and awareness take forest research to new heights? Trees For. People 1:100005. doi: 10.1016/j.tfp.2020.100005
Arseneau, J., Bélanger, N., Ouimet, R., Royer-Tardif, S., Bilodeau-Gauthier, S., Gendreau-Berthiaume, B., et al. (2021). Wood ash application in sugar maple stands rapidly improves nutritional status and growth at various developmental stages. For. Ecol. Manage. 489, 119062. doi: 10.1016/j.foreco.2021.119062
Asner, G. P., Martin, R. E., Tupayachi, R., and Llactayo, W. (2017). Conservation assessment of the Peruvian Andes and Amazon based on mapped forest functional diversity. Biol. Conserv. 210, 80–88. doi: 10.1016/j.biocon.2017.04.008
Baldocchi, D., and Collineau, S. (1994). “The physical nature of solar radiation in heterogeneous canopies: spatial and temporal attributes,” in Exploitation of Environmental Heterogeneity by Plants Ecophysiological Processes Above-and Belowground, eds M. M. Caldwell and R. W. Pearcy (San Diego, CA: Academic Press), 21–71. doi: 10.1016/B978-0-12-155070-7.50007-X
Banin, L., Feldpausch, T. R., Phillips, O. L., Baker, T., Lloyd, J., Affum-Baffoe, K., et al. (2012). What controls tropical forest architecture? Testing environmental, structural and floristic drivers: determinants of tropical forest architecture. Glob. Ecol. Biogeogr. 21, 1179–1190. doi: 10.1111/j.1466-8238.2012.00778.x
Baraloto, C., Timothy Paine, C. E., Patiño, S., Bonal, D., Hérault, B., and Chave, J. (2010). Functional trait variation and sampling strategies in species-rich plant communities. Funct. Ecol. 24, 208–216. doi: 10.1111/j.1365-2435.2009.01600.x
Baret, F., and Buis, S. (2008). “Estimating canopy characteristics from remote sensing observations: review of methods and associated problems,” in Advances in Land Remote Sensing: System, Modeling, Inversion and Application, ed. S. Liang (Dordrecht: Springer Netherlands). doi: 10.1007/978-1-4020-6450-0_7
Barker, M. G. (2002). “Rope access techniques,” in Global GCP Global Canopy Handbook: Techniques of Access and Study in the Forest Roof, eds W. Andrew and K. S. A. T. J. Mitchell (Oxford: Global Canopy Programme).
Barker, M. G. (2015). Canopy research: onwards and upwards. what’s up? Newslett. Int. Canopy Netw. 21, 1–2.
Barker, M., and Becker, P. (1995). Sap flow rate and sap nutrient content of a tropical rain forest canopy species, Dryobalanops aromatica, in Brunei. Selbyana 16, 201–211.
Barker, M. G., and Pinard, M. A. (2001). “Forest canopy research: sampling problems, and some solutions,” in Proceedings of ESF Conference: Tropical Forest Canopies: Ecology and Management, Oxford University, 12–16 December 1998, eds K. E. Linsenmair, A. J. Davis, B. Fiala, and M. R. Speight (Dordrecht: Springer Netherlands).
Barker, M. G., and Sutton, S. L. (1997). Low-tech methods for forest canopy access. Biotropica 29, 243–247. doi: 10.1111/j.1744-7429.1997.tb00032.x
Bar-Ness, Y. D., McQuillan, P. B., Whitman, M., Junker, R., Cracknell, M., and Barrows, A. (2012). Sampling forest canopy arthropod biodiversity with three novel minimal-cost trap designs. Aust. J. Entomol. 51, 12–21. doi: 10.1111/j.1440-6055.2011.00836.x
Barton, K. E., and Boege, K. (2017). Future directions in the ontogeny of plant defence: understanding the evolutionary causes and consequences. Ecol. Lett. 20, 403–411. doi: 10.1111/ele.12744
Bassow, S. L., and Bazzaz, F. A. (1997). Intra- and inter-specific variation in canopy photosynthesis in a mixed deciduous forest. Oecologia 109, 507–515. doi: 10.1007/s004420050111
Beccari, O. (1904). Wanderings in the Great Forests of Borneo. From the English Translation. London: Archibald Constable & Co. Ltd. reprinted in 1986.
Boch, S., Müller, J., Prati, D., Blaser, S., and Fischer, M. (2013). Up in the tree-the overlooked richness of bryophytes and lichens in tree crowns. PLoS One 8:e84913. doi: 10.1371/journal.pone.0084913
Bogue, R. (2016). Growth in e-commerce boosts innovation in the warehouse robot market. Ind. Rob. 43, 583–587. doi: 10.1108/IR-07-2016-0194
Bongers, F. (2001). “Methods to assess tropical rain forest canopy structure: an overview,” in Proceedings of ESF Conference: Tropical Forest Canopies: Ecology and Management, Oxford University, 12–16 December 1998, eds K. E. Linsenmair, A. J. Davis, B. Fiala, and M. R. Speight (Dordrecht: Springer Netherlands).
Botanic Gardens Conservation International [BGCI] (2021). State of the World’s Trees. Richmond: Botanic Gardens Conservation International.
Bowler, M. T., Tobler, M. W., Endress, B. A., Gilmore, M. P., and Anderson Bogue, R. (2017). Estimating mammalian species richness and occupancy in tropical forest canopies with arboreal camera traps. Remote Sens. Ecol. Conserv. 3, 146–157. doi: 10.1002/rse2.35
Brede, B., Calders, K., Lau, A., Raumonen, P., Bartholomeus, H. M., Herold, M., et al. (2019). Non-destructive tree volume estimation through quantitative structure modelling: comparing UAV laser scanning with terrestrial LIDAR. Remote Sens. Environ. 233, 111355. doi: 10.1016/j.rse.2019.111355
Cannon, C. H. (2012). A curiosity moment for tropical biology? Science 338, 467–467. doi: 10.1126/science.338.6106.467-a
Chaari, M. Z., and Al-Maadeed, S. (2021). “Chapter 18–The game of drones/weapons makers’ war on drones,” in Unmanned Aerial Systems, eds A. Koubaa and A. T. Azar (San Diego, CA: Academic Press). doi: 10.1016/B978-0-12-820276-0.00025-X
Charron, G., Robichaud-Courteau, T., La Vigne, H., Weintraub, S., Hill, A., Justice, D., et al. (2020). The DeLeaves: a UAV device for efficient tree canopy sampling. J. Unmanned Veh. Syst. 8, 245–264. doi: 10.1139/juvs-2020-0005
Chavana-Bryant, C., Malhi, Y., Anastasiou, A., Enquist, B. J., Cosio, E. G., Keenan, T. F., et al. (2019). Leaf age effects on the spectral predictability of leaf traits in Amazonian canopy trees. Sci. Total Environ. 666, 1301–1315. doi: 10.1016/j.scitotenv.2019.01.379
Chen, S.-C., Cannon, C. H., Kua, C.-S., Liu, J. J., and Galbraith, D. W. (2014). Genome size variation in the Fagaceae and its implications for trees. Tree Genet. Genomes 10, 977–988. doi: 10.1007/s11295-014-0736-y
Ciocca, D. R., and Delgado, G. (2017). The reality of scientific research in Latin America; an insider’s perspective. Cells Stress Chaperones 22, 847–852. doi: 10.1007/s12192-017-0815-8
Coley, P. D., and Barone, J. A. (1996). Herbivory and plant defenses in tropical forests. Annu. Rev. Ecol. Syst. 27, 305–335. doi: 10.1146/annurev.ecolsys.27.1.305
Corner, J. K. (2013). My Father in His Suitcase: In Search of E.J.H. Corner the Relentless Botanist. Singapore: Landmark Books.
Crazzolara, C., Ebner, M., Platis, A., Miranda, T., Bange, J., and Junginger, A. (2019). A new multicopter-based unmanned aerial system for pollen and spores collection in the atmospheric boundary layer. Atmos. Meas. Tech. 12, 1581–1598. doi: 10.5194/amt-12-1581-2019
Dainelli, R., Toscano, P., Di Gennaro, S. F., and Matese, A. (2021). Recent advances in unmanned aerial vehicles forest remote sensing—a systematic review. Part II: research applications. For. Trees Livelihoods 12:397. doi: 10.3390/f12040397
Dalponte, M., Ørka, H. O., Ene, L. T., Gobakken, T., and Næsset, E. (2014). Tree crown delineation and tree species classification in boreal forests using hyperspectral and ALS data. Remote Sens. Environ. 140, 306–317. doi: 10.1016/j.rse.2013.09.006
Davies-Colley, R. J., Payne, G. W., and van Elswijk, M. (2000). Microclimate gradients across a forest edge. New Zealand J. Ecol. 24, 111–121.
Degtjarenko, P., Mark, K., Moisejevs, R., Himelbrant, D., Stepanchikova, I., Tsurykau, A., et al. (2020). Low genetic differentiation between apotheciate Usnea florida and sorediate Usnea subfloridana (Parmeliaceae, Ascomycota) based on microsatellite data. Fungal Biol. 124, 892–902. doi: 10.1016/j.funbio.2020.07.007
Degtjarenko, P., Marmor, L., Tõrra, T., Lerch, M., Saag, A., Randlane, T., et al. (2016). Impact of alkaline dust pollution on genetic varation of Usnea subfloridana populations. Fungal Biol. 120, 1165–1174. doi: 10.1016/j.funbio.2016.05.010
Dial, R. J., Ellwood, M. D. F., Turner, E. C., and Foster, W. A. (2006). Arthropod abundance, canopy structure, and microclimate in a Bornean lowland tropical rain forest. Biotropica 38, 643–652.
Di Cerbo, A. R., and Biancardi, C. M. (2013). Monitoring small and arboreal mammals by camera traps: effectiveness and applications. Acta Theriol. 58, 279–283. doi: 10.1007/s13364-012-0122-9
Dorrington, G. E. (2005). Development of an airship for tropical rain forest canopy exploration. Aeronaut. J. 109, 361–372. doi: 10.1017/S0001924000000798
Ducrettet, M., Forget, P.-M., Ulloa, J. S., Yguel, B., Gaucher, P., Princé, K., et al. (2020). Monitoring canopy bird activity in disturbed landscapes with automatic recorders: a case study in the tropics. Biol. Conserv. 245:108574. doi: 10.1016/j.biocon.2020.108574
Ehbrecht, M., Seidel, D., Annighöfer, P., Kreft, H., Köhler, M., Zemp, D. C., et al. (2021). Global patterns and climatic controls of forest structural complexity. Nat. Commun. 12:519. doi: 10.1038/s41467-020-20767-z
Ellis, C. J. (2012). Lichen epiphyte diversity: a species, community and trait-based review. Perspect. Plant Ecol. Evol. Syst. 14, 131–152. doi: 10.1016/j.ppees.2011.10.001
Endara, M.-J., Coley, P. D., Ghabash, G., Nicholls, J., Dexter, K. G., Donoso, D. A., et al. (2017). Coevolutionary arms race versus host defense chase in a tropical herbivore–plant system. Proc. Natl. Acad. Sci. U.S.A. 114, E7499–E7505. doi: 10.1073/pnas.1707727114
Erwin, T. L. (1982). Tropical forests: their richness in coleoptera and other Arthropod Species. Coleopt. Bull. 36, 74–75.
Erwin, T. L., and Scott, J. C. (1980). Seasonal and size patterns, trophic structure, and richness of Coleoptera in the tropical arboreal ecosystem: the fauna of the tree Luehea seemannii Triana and Planch in the Canal Zone of Panama. Coleopt. Bull. 34, 305–322.
Falen Horna, L. Y., and Honorio Coronado, E. N. (2018). Assessment of the techniques use to harvest buriti fruits (Mauritia flexuosa l.f.) in the district of jenaro herrera, Loreto, Peru. Folia Amazón. 27, 131–150. doi: 10.24841/fa.v27i2.443
Falster, D. S., Duursma, R. A., and FitzJohn, R. G. (2018). How functional traits influence plant growth and shade tolerance across the life cycle. Proc. Natl. Acad. Sci. U.S.A. 115, E6789–E6798. doi: 10.1073/pnas.1714044115
Fanning, E., Ely, J. S., Thorsten Lumbsch, H., and Keller, H. W. (2007). Vertical distribution of lichen growth forms in tree canopies of great smoky Mountains National Park. Sena 6, 83–88. doi: 10.1656/1528-7092(2007)6[83:VDOLGF]2.0.CO;2
Farinha, A., Zufferey, R., Zheng, P., Armanini, S. F., and Kovac, M. (2020). Unmanned aerial sensor placement for cluttered environments. IEEE Robot. Autom. Lett. 5, 6623–6630. doi: 10.1109/LRA.2020.3015459
Feng, L., Chen, S., Zhang, C., Zhang, Y., and He, Y. (2021). A comprehensive review on recent applications of unmanned aerial vehicle remote sensing with various sensors for high-throughput plant phenotyping. Comput. Electron. Agric. 182:106033. doi: 10.1016/j.compag.2021.106033
Ferreira, M. P., Almeida, D. R. A., Papa, D., Minervino, J. B. S., Veras, H. F. P., Formighieri, A., et al. (2020). Individual tree detection and species classification of Amazonian palms using UAV images and deep learning. For. Ecol. Manage. 475:118397. doi: 10.1016/j.foreco.2020.118397
Finžgar, D., Bajc, M., Brezovar, J., Kladnik, A., Capuder, R., and Kraigher, H. (2016). Development of a patented unmanned aerial vehicle-based system for tree canopy sampling. Folia Biol. Geol. 57, 35–39. doi: 10.3986/fbg0009
Fritz, Ö (2008). Vertical distribution of epiphytic bryophytes and lichens emphasizes the importance of old beeches in conservation. Biodivers. Conserv. 18, 289–304. doi: 10.1007/s10531-008-9483-4
Ganivet, E., and Bloomberg, M. (2019). Towards rapid assessments of tree species diversity and structure in fragmented tropical forests: a review of perspectives offered by remotely-sensed and field-based data. For. Ecol. Manage. 432, 40–53. doi: 10.1016/j.foreco.2018.09.003
Gasparyan, A., Sipman, H. J. M., Marini, L., and Nascimbene, J. (2018). The inclusion of overlooked lichen microhabitats in standardized forest biodiversity monitoring. Lichenologist 50, 231–237. doi: 10.1017/S0024282918000087
Gini, R., Passoni, D., Pinto, L., and Sona, G. (2014). Use of unmanned aerial systems for multispectral survey and tree classification: a test in a park area of northern Italy. Eur. J. Remote Sens. 47, 251–269. doi: 10.5721/EuJRS20144716
Gouveia, S. F., Villalobos, F., Dobrovolski, R., Beltrão-Mendes, R., and Ferrari, S. F. (2014). Forest structure drives global diversity of primates. J. Anim. Ecol. 83, 1523–1530. doi: 10.1111/1365-2656.12241
Gregory, T., Carrasco Rueda, F., Deichmann, J., Kolowski, J., and Alonso, A. (2014). Arboreal camera trapping: taking a proven method to new heights. Methods Ecol. Evol. 5, 443–451. doi: 10.1111/2041-210X.12177
Haggerty, D. A., Naclerio, N., and Hawkes, E. W. (2021). Hybrid vine robot with internal steering-reeling mechanism enhances system-level capabilities. IEEE Robot. Autom. Lett. 6, 5437–5444. doi: 10.1109/LRA.2021.3072858
Hallé, F., Cleyet-Marrel, D., and Ebersolt, G. (2000). Le Rafeau des Cimes : Exploring Forest Canopies. Paris: JC Lattès.
Hang, K., Lyu, X., Song, H., Stork, J. A., Dollar, A. M., Kragic, D., et al. (2019). Perching and resting—a paradigm for UAV maneuvering with modularized landing gears. Sci. Robot. 4:eaau6637. doi: 10.1126/scirobotics.aau6637
Hurlbert, S. H. (1984). Pseudoreplication and the design of ecological field experiments. Ecol. Monogr. 54, 187–211. doi: 10.2307/1942661
Jarrín-V, P., Falconí, F., Cango, P., and Ramos-Martin, J. (2021). Knowledge gaps in Latin America and the Caribbean and economic development. World Dev. 146:105602. doi: 10.1016/j.worlddev.2021.105602
Jepson, J. (2000). The Tree Climber’s Companion: A Reference And Training Manual For Professional Tree Climbers. Santa Barbara, CA: Beaver Tree Pub.
Jordano, P., García, C., Godoy, J. A., and García-Castaño, J. L. (2007). Differential contribution of frugivores to complex seed dispersal patterns. PNAS 104, 3278–3282.
Junda, J., Greene, E., and Bird, D. M. (2015). Proper flight technique for using a small rotary-winged drone aircraft to safely, quickly, and accurately survey raptor nests. J. Unmanned Veh. Syst. 3, 222–236. doi: 10.1139/juvs-2015-0003
Kalantari, A., and Spenko, M. (2014). Modeling and performance assessment of the HyTAQ, a Hybrid Terrestrial/Aerial Quadrotor. IEEE Trans. Robot. 30, 1278–1285. doi: 10.1109/TRO.2014.2337555
Kapos, V., Ganade, G., Matsui, E., and Victoria, R. L. (1993). ∂ 13 C as an indicator of edge effects in tropical rainforest reserves. J. Ecol. 81, 425–432. doi: 10.2307/2261521
Kardan, O., Gozdyra, P., Misic, B., Moola, F., Palmer, L. K., Paus, T., et al. (2015). Neighborhood greenspace and health in a large urban center. Sci. Rep. 5:11610. doi: 10.1038/srep11610
Käslin, F., Baur, T., Meier, P., Koller, P., Buchmann, N., D’Odorico, P., et al. (2018). Novel twig sampling method by unmanned aerial vehicle (UAV). Front. For. Glob. Chang. 1:2. doi: 10.3389/ffgc.2018.00002
Kaufmann, S., Weinrich, T., Hauck, M., and Leuschner, C. (2019). Vertical variation in epiphytic cryptogam species richness and composition in a primeval Fagus sylvatica forest. J. Veg. Sci. 30, 881–892. doi: 10.1111/jvs.12775
Kelly, D., and Sork, V. L. (2002). Mast seeding in perennial plants: why, how, where? Annu. Rev. Ecol. Syst. 33, 427–447. doi: 10.1146/annurev.ecolsys.33.020602.095433
Kettle, C. J., Maycock, C. R., Ghazoul, J., Hollingsworth, P. M., Khoo, E., Sukri, R. S. H., et al. (2011). Ecological implications of a flower size/number trade-off in tropical forest trees. PLoS One 6:e16111. doi: 10.1371/journal.pone.0016111
Kiebacher, T., Keller, C., Scheidegger, C., and Bergamini, A. (2016). Hidden crown jewels: the role of tree crowns for bryophyte and lichen species richness in sycamore maple wooded pastures. Biodivers. Conserv. 25, 1605–1624. doi: 10.1007/s10531-016-1144-4
Klaptocz, A., Briod, A., Daler, L., Zufferey, J. C., and Floreano, D. (2013). “Euler spring collision protection for flying robots,” in Proceedings of the 2013 IEEE/RSJ International Conference on Intelligent Robots and Systems, Tokyo. doi: 10.1109/IROS.2013.6696606
Koch, G. W., Amthor, J. S., and Goulden, M. L. (1994). Diurnal patterns of leaf photosynthesis, conductance and water potential at the top of a lowland rain forest canopy in Cameroon: measurements from the Radeau des Cimes. Tree Physiol. 14, 347–360. doi: 10.1093/treephys/14.4.347
Kutia, J. (2019). Aerial manipulation for canopy sampling. (Doctoral dissertation, ResearchSpace@ Auckland).
Lam, T. L., and Xu, Y. (2011). “A flexible tree climbing robot: treebot–design and implementation,” in Proceedings of the 2011 IEEE International Conference on Robotics and Automation, Shanghai. doi: 10.1109/ICRA.2011.5979833
Lambert, T. D., Malcolm, J. R., and Zimmerman, B. L. (2005). Variation in small mammal species richness by trap height and trap type in Southeastern Amazonia. J. Mammal. 86, 982–990. doi: 10.1644/1545-1542(2005)86[982:VISMSR]2.0.CO;2
Langen, T. A., Cannon, C. H., Blackburn, D. C., Morgan, E., and Mera, P. (2021). Discovering and applying the urban rules of life to design sustainable and healthy cities. Integr. Comp. Biol. icab065. doi: 10.1093/icb/icab065
La Vigne, H., Charron, G., Hovington, S., and Lussier Desbiens, A. (2021). Assisted Canopy Sampling Using Unmanned Aerial Vehicles (UAVs), International Conference on Unmanned Aircraft Systems.
Lechner, A. M., Foody, G. M., and Boyd, D. S. (2020). Applications in remote sensing to forest ecology and management. One Earth 2, 405–412. doi: 10.1016/j.oneear.2020.05.001
Leponce, M., Corbara, B., and Basset, Y. (2012). “IBISCA–a collaborative programme to study the diversity and distribution of arthropods from canopy to forest floor,” in Forest Canopy Methods, eds M. D. Lowman, T. Schowalter, and J. Franklin (Berkeley, CA: University of California Press), 34–39.
Leponce, M., Dejean, A., Mottl, O., and Klimes, P. (2021). Rapid assessment of the three-dimensional distribution of dominant arboreal ants in tropical forests. Insect Conserv. Divers. 14, 426–438. doi: 10.1111/icad.12486
Löcken, H., Fischer, O. W., Selz, J., and Boppré, M. (2020). ‘Drone-netting’ for sampling live insects. J. Insect Sci. 20, 1–3.
Longo, D., Caruso, L., Conti, A., Camillieri, D., and Schillaci, G. (2013). A survey of safety issues in tree-climbing applications for forestry management. J. Agric. Eng. Res. 44:e141. doi: 10.4081/jae.2013.383
Lowman, M. D. (2001). Plants in the forest canopy: some reflections on current research and future direction. Plant Ecol. 153, 39–50.
Lowman, M. (2021). Life in the treetops—an overview of forest canopy science and its future directions. Plants People Planet 3, 16–21. doi: 10.1002/ppp3.10125
Lowman, M. D., and Bouricius, B. (1995). The construction of platforms and bridges for forest canopy access. Selbyana 16, 179–184.
Lowman, M. D., Schowalter, T. D., and Franklin, J. (2012). Methods in Forest Canopy Research. Berkeley, CA: University of California Press. doi: 10.1525/9780520953925
Makarieva, A. M., Gorshkov, V. G., Sheil, D., Nobre, A. D., Bunyard, P., and Li, B.-L. (2014). Why does air passage over forest yield more rain? Examining the coupling between rainfall, pressure, and atmospheric moisture content. J. Hydrometeorol. 15, 411–426. doi: 10.1175/JHM-D-12-0190.1
Marks, W. L., Iiames, J. S., Lunetta, R. S., Khorram, S., and Mace, T. H. (2014). Basal area and biomass estimates of loblolly pine stands using L-band UAVSAR. Photogramm. Eng. Remote Sensing 80, 33–42. doi: 10.14358/PERS.80.1.33
Marmor, L., Torra, T., Lauri, S., Leppik, E., and Randlane, T. (2013). Lichens on Picea abies and Pinus sylvestris-from tree bottom to the top. Lichenologist 45, 51. doi: 10.1017/S0024282912000564
Matsler, A. M., Meerow, S., Mell, I. C., and Pavao-Zuckerman, M. A. (2021). A ‘green’ chameleon: Exploring the many disciplinary definitions, goals, and forms of “green infrastructure.”. Landsc. Urban Planning 214L:104145. doi: 10.1016/j.landurbplan.2021.104145
McCaig, T., Sam, L., Nakamura, A., and Stork, N. E. (2020). Is insect vertical distribution in rainforests better explained by distance from the canopy top or distance from the ground? Biodivers. Conserv. 29, 1081–1103. doi: 10.1007/s10531-019-01927-0
McClearn, D., Kohler, J., McGowan, K. J., Cedeno, E., Carbone, L. G., and Miller, D. (1994). Arboreal and terrestrial mammal trapping on Gigante Peninsula, Barro Colorado Nature Monument, Panama. Biotropica 26, 208–213. doi: 10.2307/2388810
McDowell, N., Allen, C. D., Anderson-Teixeira, K., Brando, P., Brienen, R., Chamber, J., et al. (2018). Drivers and mechanisms of tree mortality in moist tropical forests. New Phytol. 219, 851–869. doi: 10.1111/nph.15027
Megalingam, R. K., Manoharan, S. K., Mohandas, S. M., Vadivel, S. R. R., Ganggireddy, R., Ghanta, S., et al. (2021). Amaran: an unmanned robotic coconut tree climber and harvester. IEEE/ASME Trans. Mechatron. 26, 288–299.
Mehanovic, D., Rancourt, D., and Desbiens, A. L. (2019). Fast and efficient aerial climbing of vertical surfaces using fixed-wing UAVs. IEEE Robot. Autom. Lett. 4, 97–104. doi: 10.1109/LRA.2018.2881433
Milne, S., Martin, J. G. A., Reynolds, G., Vairappan, C. S., Slade, E. M., Brodie, J. F., et al. (2021). Drivers of bornean orangutan distribution across a multiple-use tropical landscape. Remote Sensing 13:458. doi: 10.3390/rs13030458
Mirallès, F., Hamelin, P., Lambert, G., Lavoie, S., Pouliot, N., Montfrond, M., et al. (2018). “LineDrone technology: landing an unmanned aerial vehicle on a power line,” in Proceedings of the 2018 IEEE International Conference on Robotics and Automation (ICRA), Brisbane, QLD. doi: 10.1109/ICRA.2018.8461250
Mitchell, A. (2001). “Introduction — canopy science: time to shape up,” in Tropical Forest Canopies: Ecology and Management, eds K. E. Linsenmair, A. J. Davis, B. Fiala, and M. R. Speight (Dordrecht: Springer), 5–11. doi: 10.1007/978-94-017-3606-0_1
Mitchell, A. W. (1986). The Enchanted Canopy: Secrets from the Rainforest Roof. New York, NY: HarperCollins.
Mitchell, A. W., Secoy, K., and Jackson, T. (2002). The Global Canopy Handbook: Techniques of Access and Study in the Forest Roof. Oxford: Global Canopy Programme.
Moffett, M. W. (1993). The High Frontier: Exploring the Tropical Rainforest Canopy. Cambridge, MA: Harvard University Press.
Moffett, W. M., and Lowman, M. D. (1995). “Canopy access techniques,” in Forest Canopies, eds M. D. Lowman and N. M. Nadkarni (San Diego, CA: Academic Press), 3–26.
Mottl, O., Fibich, P., Klimes, P., Volf, M., Tropek, R., Anderson-Teixeira, K., et al. (2020). Spatial covariance of herbivorous and predatory guilds of forest canopy arthropods along a latitudinal gradient. Ecol. Lett. 23, 1499–1510. doi: 10.1111/ele.13579
Nadkarni, N. M., Parker, G. G., and Lowman, M. D. (2011). Forest canopy studies as an emerging field of science. Ann. For. Sci. 68:217. doi: 10.1007/s13595-011-0046-6
Nadkarni, N. M., Parker, G. G., Ford, E. D., and Stallman, C. (1996). The international canopy network: a pathway for interdisciplinary exchange of scientific information on forest canopies. Northwest Sci. 70, 104–108.
Nakamura, A., Kitching, R. L., Cao, M., Creedy, T. J., Fayle, T. M., Freiberg, M., et al. (2017). Forests and their canopies: achievements and horizons in canopy science. Trends Ecol. Evol. 32, 438–451. doi: 10.1016/j.tree.2017.02.020
Nazir, S., and Kaleem, M. (2021). Advances in image acquisition and processing technologies transforming animal ecological studies. Ecol. Inform. 61:101212. doi: 10.1016/j.ecoinf.2021.101212
NEON (2019). Going Out on a Limb with a New Canopy Sampling Method, NEON Sciences. Available online at: https://www.neonscience.org/impact/observatory-blog/going-out-limb-new-canopy-sampling-method
Newmark, W. D. (2001). Tanzanian forest edge microclimatic gradients: Dynamic Patterns. Biotropica 33, 2–11.
Nguyen, H.-N., Siddall, R., Stephens, B., Navarro-Rubio, A., and Kovač, M. (2019). “A Passively adaptive microspine grapple for robust, controllable perching,” in Proceedings of the 2019 2nd IEEE International Conference on Soft Robotics (RoboSoft), Seoul. doi: 10.1109/ROBOSOFT.2019.8722779
Nitta, J. H., Watkins, J. E. Jr., and Davis, C. C. (2020). Life in the canopy: community trait assessments reveal substantial functional diversity among fern epiphytes. New Phytol. 227, 1885–1899. doi: 10.1111/nph.16607
Nowak, D. J., Hirabayashi, S., Bodine, A., and Greenfield, E. (2014). Tree and forest effects on air quality and human health in the United States. Environ. Pollut. 193, 119–129. doi: 10.1016/j.envpol.2014.05.028
Oliveira, B. F., and Scheffers, B. R. (2019). Vertical stratification influences global patterns of biodiversity. Ecography 42, 249–249. doi: 10.1111/ecog.03636
Ozanne, C. M. P., Anhuf, D., Boulter, S. L., Keller, M., Kitching, R. L., Körner, C., et al. (2003). Biodiversity meets the atmosphere: a global view of forest canopies. Science 301, 183–186. doi: 10.1126/science.1084507
Parker, G. G., Lowman, M. D., and Nadkarni, N. M. (1995). For Canopies. San Diego, CA: Academic Press.
Pearse, I. S., LaMontagne, J. M., Lordon, M., Hipp, A. L., and Koenig, W. D. (2020). Biogeography and phylogeny of masting: do global patterns fit functional hypotheses? New Phytol. 227, 1557–1567. doi: 10.1111/nph.16617
Pérez-Granados, C., and Traba, J. (2021). Estimating bird density using passive acoustic monitoring: a review of methods and suggestions for further research. IBIS 163, 765–783. doi: 10.1111/ibi.12944
Perez-Salicrup, D. R., Claros, A., Guzman, R., Licona, J. C., Ledezma, F., Pinard, M. A., et al. (2001). Cost and efficiency of cutting lianas in a lowland Liana forest of Bolivia1. Biotropica 33, 324–329. doi: 10.1111/j.1744-7429.2001.tb00183.x
Perry, D. R. (1978). A method of access into the crowns of emergent and canopy trees. Biotropica 10, 155–157. doi: 10.2307/2388019
Perry, D. R., and Williams, J. (1981). The tropical rain forest canopy: a method providing total access. Biotropica 13, 283–285. doi: 10.2307/2387806
Petter, G., Wagner, K., Wanek, W., Delgado, E. J. S., Zotz, G., Cabral, J. S., et al. (2016). Functional leaf traits of vascular epiphytes: vertical trends within the forest, intra-and interspecific trait variability, and taxonomic signals. Funct. Ecol. 30, 188–198. doi: 10.1111/1365-2435.12490
Rinker, H. B., and Jarzen, D. M. (2004). “Reintegration of wonder into the emerging science of canopy ecology,” in Forest Canopies, eds M. D. Lowman and H. B. Rinker (San Diego, CA: Elsevier). doi: 10.1016/B978-012457553-0/50033-2
Roderick, W. R. T., Cutkosky, M. R., and Lentink, D. (2017). Touchdown to take-off: at the interface of flight and surface locomotion. Interface Focus 7:20160094. doi: 10.1098/rsfs.2016.0094
Santiago, L. S., Kitajima, K., Wright, S. J., and Mulkey, S. S. (2004). Coordinated changes in photosynthesis, water relations and leaf nutritional traits of canopy trees along a precipitation gradient in lowland tropical forest. Oecologia 139, 495–502.
Sarmento Cabral, J., Petter, G., Mendieta-Leiva, G., Wagner, K., Zotz, G., and Kreft, H. (2015). Branchfall as a demographic filter for epiphyte communities: lessons from forest floor-based sampling. PLoS One 10:e0128019. doi: 10.1371/journal.pone.0128019
Scheffers, B. R., Phillips, B. L., Laurance, W. F., Sodhi, N. S., Diesmos, A., and Williams, S. E. (2013). Increasing arboreality with altitude: a novel biogeographic dimension. Proc. Biol. Sci. 280:20131581. doi: 10.1098/rspb.2013.1581
Scher, C. L., Griffoul, E., and Cannon, C. H. (2019). Drone-based photogrammetry for the construction of high-resolution models of individual trees. Trees 33, 1385–1397. doi: 10.1007/s00468-019-01866-x
Schlesinger, W. H., and Jasechko, S. (2014). Transpiration in the global water cycle. Agric. For. Meteorol. 189–190, 115–117. doi: 10.1016/j.agrformet.2014.01.011
Schweiger, A. K., Desbiens, A. L., Charron, G., La Vigne, H., and Laliberté, E. (2020). Foliar sampling with an unmanned aerial system (UAS) reveals spectral and functional trait differences within tree crowns. Can. J. For. Res. 50, 966–974. doi: 10.1139/cjfr-2019-0452
Sedio, B. E. (2017). Recent breakthroughs in metabolomics promise to reveal the cryptic chemical traits that mediate plant community composition, character evolution and lineage diversification. New Phytol. 214, 952–958. doi: 10.1111/nph.14438
Seidler, T. G., and Plotkin, J. B. (2006). Seed dispersal and spatial pattern in tropical trees. PLoS Biol. 4:e344.
Shenkin, A., Chandler, C. J., Boyd, D. S., Jackson, T., Disney, M., Majalap, N., et al. (2019). The world’s tallest tropical tree in three dimensions. Front. For. Glob. Chang. 2:32. doi: 10.3389/ffgc.2019.00032
Slik, J. W. F., Paoli, G., McGuire, K., Amaral, L., Barroso, J., Bastian, M., et al. (2013). Large trees drive forest aboveground biomass variation in moist lowland forests across the tropics. Glob. Ecol. Biogeogr. 22, 1261–1271. doi: 10.1111/geb.12092
Spaan, D., Burke, C., McAree, O., Aureli, F., Rangel-Rivera, C. E., Hutschenreiter, A., et al. (2019). Thermal infrared imaging from drones offers a major advance for spider monkey surveys. Drones 3:34. doi: 10.3390/drones3020034
Sutton, S. L. (2001). “Alice grows up: canopy science in transition from Wonderland to Reality,” in Proceedings of ESF Conference: Tropical Forest Canopies: Ecology and Management, Oxford University, 12–16 December 1998, eds K. E. Linsenmair, A. J. Davis, B. Fiala, and M. R. Speight (Dordrecht: Springer Netherlands).
Swart, R. C., Samways, M. J., and Roets, F. (2020). Tree canopy arthropods have idiosyncratic responses to plant ecophysiological traits in a warm temperate forest complex. Sci. Rep. 10:19905. doi: 10.1038/s41598-020-76868-8
Tagle Casapia, X., Falen, L., Bartholomeus, H., Cárdena, R., Flores, G., Herold, M., et al. (2019). Identifying and quantifying the abundance of economically important palms in tropical moist forest using UAV imagery. Remote Sensing 12:9. doi: 10.3390/rs12010009
Taylor, N. P. (2019). E.J.H. Corner—Mabberley’s mentor—and his contributions to the Singapore Botanic Gardens’ heritage. Gard. Bull. Singapore 71, 47–52. doi: 10.26492/gbs71(suppl.2).2019-06
Thomson, E. R., Malhi, Y., Bartholomeus, H., Oliveras, I., Gvozdevaite, A., Paprah, T., et al. (2018). Mapping the leaf economic spectrum across West African tropical forests using UAV-acquired hyperspectral imagery. Remote Sensing 10:1532. doi: 10.3390/rs10101532
Trammell, T. L. E., D’Amico, V. I. I. I., Avolio, M. L., Mitchell, J. C., and Moore, E. (2020). Temperate deciduous forests embedded across developed landscapes: younger forests harbour invasive plants and urban forests maintain native plants. J. Ecol. 108, 2366–2375. doi: 10.1111/1365-2745.13400
Turner-Skoff, J. B., and Cavender, N. (2019). The benefits of trees for livable and sustainable communities. Plants People Planet 1, 323–335. doi: 10.1002/ppp3.39
Ulrich, R. S. (1984). View through a window may influence recovery from surgery. Science 224, 420–421. doi: 10.1126/science.6143402
van Dulmen, A. (2001). “Pollination and phenology of flowers in the canopy of two contrasting rain forest types in Amazonia, Colombia,” in Tropical Forest Canopies: Ecology and Management. Forestry Sciences, eds K. E. Linsenmair, A. J. Davis, B. Fiala, and M. R. Speight (Dordrecht: Springer), 69. doi: 10.1007/978-94-017-3606-0_7
Volf, M., Klimeš, P., Lamarre, G. P. A., Redmond, C. M., Sifert, C. L., Abe, T., et al. (2019). Quantitative assessment of plant-arthropod interactions in forest canopies: a plot-based approach. PLoS One 14:e0222119. doi: 10.1371/journal.pone.0222119
Wagner, F. H., Dalagnol, R., Tagle Casapia, X., Annia, S. S., Oliver, F. P., Emanuel, G., et al. (2020). Regional mapping and spatial distribution analysis of canopy palms in an amazon forest using deep learning and VHR images. Remote Sensing 12:2225. doi: 10.3390/rs12142225
Wallace, A. R. (1869). The Malay Archipelago: The Land of the Orang–Utan, and the Bird of Paradise. A Narrative of Travel, with Sketches of Man and Nature. London: Macmillan. doi: 10.5962/bhl.title.134833
Wang, X., Wang, Y., Zhou, C., Yin, L., and Feng, X. (2021). Urban forest monitoring based on multiple features at the single tree scale by UAV. Urban For. Urban Green. 58:126958. doi: 10.1016/j.ufug.2020.126958
Weishampel, J. F., Ranson, K. J., and Harding, D. J. (1996). Remote sensing of forest canopies. Selbyana 17, 6–14.
Whitworth, A., Braunholtz, L. D., Huarcaya, R. P., MacLeod, R., and Beirne, C. (2016). Out on a limb: arboreal camera traps as an emerging methodology for inventorying elusive rainforest mammals. Trop. Conserv. Sci. 9, 675–698. doi: 10.1177/194008291600900208
Yaacoub, J.-P., Noura, H., Salman, O., and Chehab, A. (2020). Security analysis of drones systems: attacks, limitations, and recommendations. Internet Things 11:100218. doi: 10.1016/j.iot.2020.100218
Zandt, H. S. (1994). A comparison of three sampling techniques to estimate the population size of caterpillars in trees. Oecologia 97, 399–406. doi: 10.1007/BF00317331
Zhang, H., Wang, C., Turvey, S. T., Sun, Z., Tan, Z., Yang, Q., et al. (2020). Thermal infrared imaging from drones can detect individuals and nocturnal behavior of the world’s rarest primate. Glob. Ecol. Conserv. 23:e01101. doi: 10.1016/j.gecco.2020.e01101
Keywords: canopy crane, tree climbing, robotics, epiphytic, arbornaut, sampling design, canopy access, canopy biology, Drones (UAV)
Citation: Cannon CH, Borchetta C, Anderson DL, Arellano G, Barker M, Charron G, LaMontagne JM, Richards JH, Abercrombie E, Banin LF, Tagle Casapia X, Chen X, Degtjarenko P, Dell JE, Durden D, Guevara Andino JE, Hernández-Gutiérrez R, Hirons AD, Kua C-S, La Vigne H, Leponce M, Lim JY, Lowman M, Marshall AJ, Michaletz ST, Normark BB, Penneys DS, Schneider GF, Strijk JS, Tiamiyu BB, Trammell TLE, Vargas-Rodriguez YL, Weintraub-Leff SR, Lussier Desbiens A and Spenko M (2021) Extending Our Scientific Reach in Arboreal Ecosystems for Research and Management. Front. For. Glob. Change 4:712165. doi: 10.3389/ffgc.2021.712165
Received: 20 May 2021; Accepted: 04 October 2021;
Published: 08 November 2021.
Edited by:
Brett Scheffers, University of Florida, United StatesReviewed by:
Romà Ogaya, Ecological and Forestry Applications Research Center (CREAF), SpainLily Leahy, James Cook University, Australia
Copyright © 2021 Cannon, Borchetta, Anderson, Arellano, Barker, Charron, LaMontagne, Richards, Abercrombie, Banin, Tagle Casapia, Chen, Degtjarenko, Dell, Durden, Guevara Andino, Hernández-Gutiérrez, Hirons, Kua, La Vigne, Leponce, Lim, Lowman, Marshall, Michaletz, Normark, Penneys, Schneider, Strijk, Tiamiyu, Trammell, Vargas-Rodriguez, Weintraub-Leff, Lussier Desbiens and Spenko. This is an open-access article distributed under the terms of the Creative Commons Attribution License (CC BY). The use, distribution or reproduction in other forums is permitted, provided the original author(s) and the copyright owner(s) are credited and that the original publication in this journal is cited, in accordance with accepted academic practice. No use, distribution or reproduction is permitted which does not comply with these terms.
*Correspondence: Charles H. Cannon, Y2Nhbm5vbkBtb3J0b25hcmIub3Jn